Brittle-Ductile Transition Depth Versus Convergence Rate in Shallow Crustal Thrust Faults: Considerations on Seismogenic Volume and Impact on Seismicity
Total Page:16
File Type:pdf, Size:1020Kb
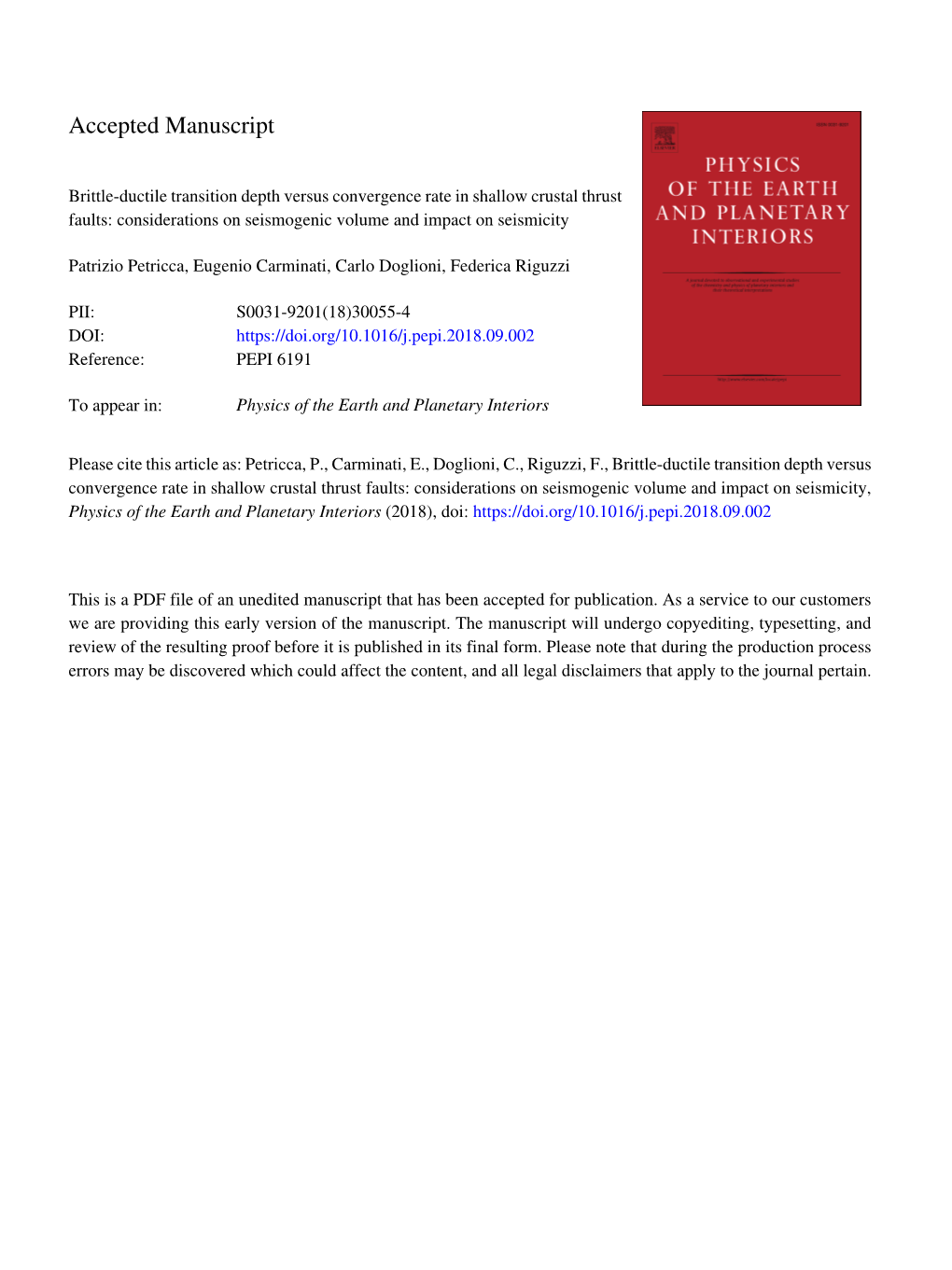
Load more
Recommended publications
-
On the Thermodynamics of Listric Faults
Earth Planets Space, 56, 1111–1120, 2004 On the thermodynamics of listric faults Klaus Regenauer-Lieb1,2, Bruce Hobbs2, and Alison Ord2 1Johannes Gutenberg-Universitat¨ Mainz, Geophysics and Geodynamics, 55099 Mainz, Germany 2CSIRO Exploration and Mining Perth (ARRC), PO Box 1130, Bentley WA 6102, Australia (Received June 15, 2004; Revised November 21, 2004; Accepted December 4, 2004) We investigate a novel fully coupled thermal-mechanical numerical model of the crust in order to trace the physics of interaction of its brittle and ductile layers. In a unified approach these layers develop in a natural transition as a function of the state variables pressure, deviatoric stress, temperature and strain-rate. We find that the main storage of elastic energy lies in the domain where brittle and ductile strain-rates overlap so that shear zones are attracted to this zone of maximum energy dissipation. This dissipation appears as a local heat source (shear heating). The brittle-ductile transition zone evolves through extreme weakening by thermo-mechanical feedback. The physics of the weakening process relies on repeated breaching of a critical energy flux threshold for feedback within this sub-horizontal brittle-ductile flow layer, thus developing unstable slipping events at post- and pre-seismic strain-rates. The width- and the temperature domain of the feedback layer is controlled by the activation enthalpy Q of the material. For olivine rheology (Q ∼ 500 kJ/mol) the layer can be extremely thin <500 m and adheres to the 875 K isotherm. For quartz (Q ∼ 135 kJ/mol) the width of the feedback layer fans out into multiple interacting ductile faults covering a temperature domain of 450–600 K. -
Variations in Wedge Earthquake Distribution Along the Strike Underlain by Thermally Controlled Hydrated Megathrusts
applied sciences Article Variations in Wedge Earthquake Distribution along the Strike Underlain by Thermally Controlled Hydrated Megathrusts Rui Qu 1,2 , Yingfeng Ji 1,2,* and Weiling Zhu 1,3,* 1 State Key Laboratory of Tibetan Plateau Earth System, Resources and Environment (TPESRE), Institute of Tibetan Plateau Research, Chinese Academy of Sciences, Beijing 100101, China; [email protected] 2 College of Earth and Planetary Sciences, University of Chinese Academy of Sciences, Beijing 100049, China 3 Department of Geophysics, Yunnan University, Kunming 650091, China * Correspondence: [email protected] (Y.J.); [email protected] (W.Z.) Featured Application: Resource and environmental effects. Abstract: Accretionary wedge earthquakes usually occur in the overriding crust close to the trench or above the cold nose of the mantle wedge. However, the mechanism and temperature properties related to the slab dip angle remain poorly understood. Based on 3D thermal models to estimate the subduction wedge plate temperature and structure, we investigate the distribution of wedge earthquakes in Alaska, which has a varying slab dip angle along the trench. The horizontal distance of wedge-earthquake hypocenters significantly increases from the Aleutian Islands to south–central Alaska due to a transition from steep subduction to flat subduction. Slab dehydration inside the subducted Pacific plate indicates a simultaneous change in the distances between the intraslab metamorphic fronts and the Alaskan Trench at various depths, which is associated with the flattening Citation: Qu, R.; Ji, Y.; Zhu, W. of the Pacific plate eastward along the strike. The across-arc width of the wedge-earthquake source Variations in Wedge Earthquake zone is consistent with the across-arc width of the surface high topography above the fully dehydrated Distribution along the Strike megathrust, and the fluid upwelling spontaneously influences wedge seismotectonics and orogenesis. -
Earthquake Cycle Deformation and the Moho: Implications for the Rheology of Continental Lithosphere
Tectonophysics 609 (2013) 504–523 Contents lists available at ScienceDirect Tectonophysics journal homepage: www.elsevier.com/locate/tecto Review Article Earthquake cycle deformation and the Moho: Implications for the rheology of continental lithosphere Tim J. Wright a,⁎,JohnR.Elliottb, Hua Wang c, Isabelle Ryder d a COMET+, School of Earth and Environment, University of Leeds, Leeds LS2 9JT, UK b COMET+, Department of Earth Sciences, University of Oxford, Oxford OX1 3AN, UK c Department of Surveying Engineering, Guangdong University of Technology, Guangzhou, China d School of Environmental Sciences, 4 Brownlow St, University of Liverpool, L69 3GP, UK article info abstract Article history: The last 20 years has seen a dramatic improvement in the quantity and quality of geodetic measurements of the Received 18 June 2012 earthquake loading cycle. In this paper we compile and review these observations and test whether crustal thick- Received in revised form 20 July 2013 ness exerts any control. We found 78 earthquake source mechanisms for continental earthquakes derived from Accepted 22 July 2013 satellite geodesy, 187 estimates of interseismic “locking depth”, and 23 earthquakes (or sequences) for which Available online 6 August 2013 postseismic deformation has been observed. Globally we estimate seismogenic thickness to be 14 ± 5 and 14 ± 7 km from coseismic and interseismic observations respectively. We find that there is no global relation- Keywords: Moho ship between Moho depth and the seismogenic layer thickness determined geodetically. We also found no Crustal deformation clear global relationship between seismogenic thickness and proxies for the temperature structure of the crust. Geodesy This suggests that the effect of temperature, so clear in oceanic lithosphere, is masked in the continents by consid- Continental rheology erable variation in lithology, strain-rate, and/or grain size. -
Seamount Subduction and Earthquakes
or collective redistirbution of any portion of this article by photocopy machine, reposting, or other means is permitted only with the approval of The approval portionthe ofwith any articlepermitted only photocopy by is of machine, reposting, this means or collective or other redistirbution This article has This been published in MOUNTAINS IN THE SEA Oceanography Seamount Subduction journal of The 23, Number 1, a quarterly , Volume and Earthquakes BY AnTHONY B. WATTS, AnTHONY A.P. KOppERS, AND DAVID P. ROBINSON O ceanography ceanography S ociety. ociety. Daiichi-Kashima © Seamount The 2010 by Japan EURASIAN Trench O PLATE ceanography A O ceanography ceanography S B ociety. Mw = 7.0 A ll rights reserved. Permission is granted to copy this article for use in teaching and research. article for use and research. this copy in teaching to granted ll rights reserved. is Permission 1982 S ociety. ociety. PACIFIC Figure 1. Composite figure illustrating S the morphology of Daiichi-Kashima or Th e [email protected] to: correspondence all end PLATE Seamount, which is about to enter the b) Japan subduction zone. (a) Schematic model. Based on Figure 2 in Mogi and Nishizawa (1980) (b) Perspective view 4 km showing the trench-parallel fault that splits the seamount vertically and offsets its once-flat top into two gently tilted 2.0 surfaces, A and B. Based on swath bathym- etry data in Lallemand, et al. (1989), 3.0 5 8 km 2.0 Dominguez et al. (1998) and http://www. 4.0 utdallas.edu/~rjstern/pdfs/TrenchProof.pdf O ceanography ceanography 5.0 5.0 The red-filled star shows the approximate 6.0 location of the 1982 Mw = 7.0 earthquake c) 10 (Mochizuki et al., 2008). -
Lithospheric Strength and Its Relationship to the Elastic and Seismogenic Layer Thickness
Available online at www.sciencedirect.com R Earth and Planetary Science Letters 213 (2003) 113^131 www.elsevier.com/locate/epsl Lithospheric strength and its relationship to the elastic and seismogenic layer thickness A.B. Watts a;Ã, E.B. Burov b a Department of Earth Sciences, University of Oxford, Parks Road, Oxford OX1 3PR, UK b Laboratoire de Tectonique UMR7072, Universite¤ Pierre et Marie Curie, 4 Place Jussieu, 75252 Paris Cedex 05, France Received 31 December 2002; received in revised form 6 May 2003; accepted 12 May 2003 Abstract Plate flexure is a phenomenon that describes how the lithosphere responds to long-term ( s 105 yr) geological loads. By comparing the flexure in the vicinity of ice, volcano, and sediment loads to predictions based on simple plate models it has been possible to estimate the effective elastic thickness of the lithosphere, Te. In the oceans, Te is the range 2^50 km and is determined mainly by plate and load age. The continents, in contrast, are characterised by Te values of up to 80 km and greater. Rheological considerations based on data from experimental rock mechanics suggest that Te reflects the integrated brittle, elastic and ductile strength of the lithosphere. Te differs, therefore, from the seismogenic layer thickness, Ts, which is indicative of the depth to which anelastic deformation occurs as unstable frictional sliding. Despite differences in their time scales, Te and Ts are similar in the oceans where loading reduces the initial mechanical thickness to values that generally coincide with the thickness of the brittle layer. They differ, however, in continents, which, unlike oceans, are characterised by a multi-layer rheology. -
Extension Systems
69 EXTENSION SYSTEMS Extension systems are zones where plates split into two or more smaller blocks that move apart. To accommodate the separation, dominantly normal faults and even open fissures lead to stretching, rupture and lengthening of crustal rocks. At the same time, the lithosphere is thinned and the asthenosphere is upwelling below the necked lithosphere. Decompression during upwelling of the mantle results in partial melting. The produced basaltic magma is injected into the fissures or extruded as fissure eruptions along and on either side of the splitting linear region (graben and rifts). This mechanism, coeval lithospheric stretching and accretion of buoyant magma, is called rifting. It is called seafloor spreading once a rifted region becomes a plate boundary that creates new oceanic lithosphere as plates diverge from one another. The spreading centres shape elevated morphological forms, the mid-oceanic ridges, because magma and young, thin oceanic lithosphere are buoyant. Divergent plate boundaries are some of the most active volcanic zones on the Earth. Seafloor spreading is so important that it has created more than half of the Earth’s surface during the past 200 Ma. Since the new continents drift away from the locus of extension, they escape further deformation and marine sedimentation seals relict structures of the early rift on either side of the new ocean. These two sides are passive continental margins. The dominant stress field is extension. Bulk lithospheric rheologies control the development of large- scale extensional -
Controls on Mid-Ocean Ridge Normal Fault Seismicity Across Spreading Rates from Rate-And-State Friction Models Hannah Mark, Mark Behn, Jean-Arthur Olive, Yajing Liu
Controls on Mid-ocean Ridge Normal Fault Seismicity Across Spreading Rates From Rate-and-State Friction Models Hannah Mark, Mark Behn, Jean-Arthur Olive, Yajing Liu To cite this version: Hannah Mark, Mark Behn, Jean-Arthur Olive, Yajing Liu. Controls on Mid-ocean Ridge Normal Fault Seismicity Across Spreading Rates From Rate-and-State Friction Models. Journal of Geophysical Research, American Geophysical Union, 2018, 10.1029/2018JB015545. hal-02395020 HAL Id: hal-02395020 https://hal.archives-ouvertes.fr/hal-02395020 Submitted on 5 Dec 2019 HAL is a multi-disciplinary open access L’archive ouverte pluridisciplinaire HAL, est archive for the deposit and dissemination of sci- destinée au dépôt et à la diffusion de documents entific research documents, whether they are pub- scientifiques de niveau recherche, publiés ou non, lished or not. The documents may come from émanant des établissements d’enseignement et de teaching and research institutions in France or recherche français ou étrangers, des laboratoires abroad, or from public or private research centers. publics ou privés. Journal of Geophysical Research: Solid Earth RESEARCH ARTICLE Controls on Mid-ocean Ridge Normal Fault Seismicity Across 10.1029/2018JB015545 Spreading Rates From Rate-and-State Friction Models Key Points: Hannah F. Mark1,2 , Mark D. Behn2 , Jean-Arthur Olive3 , and Yajing Liu4 • The seismic coupling coefficient for ridge normal faults scales with 1MIT/WHOI Joint Program in Oceanography/Applied Ocean Science and Engineering, Woods Hole, MA, USA, 2Department downdip -
Rheological Behaviour in Continental and Oceanic Subduction: Inferences for the Seismotectonics of the Aegean Region
Turkish Journal of Earth Sciences Turkish J Earth Sci (2020) 29: 381-405 http://journals.tubitak.gov.tr/earth/ © TÜBİTAK Research Article doi:10.3906/yer-1909-4 Rheological behaviour in continental and oceanic subduction: inferences for the seismotectonics of the Aegean Region 1,2 1,2, Massimiliano MAGGINI , Riccardo CAPUTO * 1 Department of Physics and Earth Sciences, University of Ferrara, Ferrara, Italy 2 Interuniversity Centre for Three-Dimensional Seismotectonics, CRUST-UR Ferrara, Ferrara, Italy Received: 04.09.2019 Accepted/Published Online: 17.12.2019 Final Version: 16.03.2020 Abstract: We reconstructed several rheological transects across the Aegean Region, comparing the behaviour in collisional versus subducting settings. We interpolated closely spaced 1D strength envelopes, realized through a dedicated MATLAB script, for determining the shallow lithospheric distribution of brittle and ductile layers. We mainly used literature data and geodynamic considerations to fix the parameters for the rheological modelling and took particular care in reproducing reliable thermal models. The results of the mechanical-rheological model highlighted the following features and differences between the northern continental collision and the southern oceanic subduction settings: i) a slightly shallower brittle-ductile transition (BDT) in the western sectors of the northern transects (~30–33 km) with respect to the southern ones (~40 km); ii) on the contrary, in the central-eastern sectors of the investigated area, corresponding to an extensional tectonic regime, the northern transects have a relatively deeper BDT (about 20–25 km) compared with the southern ones (about 15 km); iii) the occurrence of a thick, deeper brittle layer below the shallowest BDT, in the central- eastern sectors of the northern transects. -
Variations of the Lithospheric Strength and Elastic Thickness in North America
Originally published as: Tesauro, M., Kaban, M. K., Mooney, W. D. (2015): Variations of the lithospheric strength and elastic thickness in North America. - Geochemistry Geophysics Geosystems (G3), 16, 7, p. 2197-2220. DOI: http://doi.org/10.1002/2015GC005937 PUBLICATIONS Geochemistry, Geophysics, Geosystems RESEARCH ARTICLE Variations of the lithospheric strength and elastic thickness 10.1002/2015GC005937 in North America Key Points: Magdala Tesauro1,2, Mikhail K. Kaban2, and Walter D. Mooney3 We estimate strength and Te distribution of the North American 1Department of Earth Sciences, Utrecht University, Utrecht, Netherlands, 2Helmholtz-Zentrum Potsdam, continent Deutsches GeoForschungsZentrum GFZ, Germany, 3U.S. Geological Survey, Menlo Park, California, USA Thermal more than compositional changes induce main strength and Te variations Seismicity is concentrated where Abstract We evaluate the effect of temperature variations on strength and effective elastic thickness (Te) large contrast of strength is of the lithosphere of the North American (NA) continent. To this purpose, we use two thermal models that estimated are corrected for compositional variations and anelasticity effects in the upper mantle. These thermal mod- els are obtained from a joint inversion of gravity data and two recent seismic tomography models (NA07 Supporting Information: and SL2013sv). The crustal rheology was defined using NACr14, the most recent NA crustal model. This Supporting Information S1 model specifies seismic velocities and thickness for a three-layer model of the crystalline crust. Strength in the lithosphere and in the crust has similar distributions, indicating that local geotherms play a dominant Correspondence to: M. Tesauro, role in determining strength rather than crustal composition. A pronounced contrast is present in strength [email protected] between cratonic and off-cratonic regions. -
Widening of the Andes: an Interplay Between Subduction Dynamics and Crustal Wedge Tectonics
Revised version – accepted in Earth Science Reviews – march 2020 Widening of the Andes: an interplay between subduction dynamics and crustal wedge tectonics Joseph Martinod(1), Mélanie Gérault(2), Laurent Husson(3), Vincent Regard (4) (1) ISTerre, Université Grenoble Alpes, Université de Savoie Mont Blanc, CNRS, IRD, IFSTTAR, 38000 Grenoble, France. [email protected] (2) Department of Earth, Atmospheric and Planetary Sciences, Massachusetts Institute of Technology, Cambridge, MA 02139, U.S.A. (3) Géosciences Environnement Toulouse, Université de Toulouse, CNRS, UPS(OMP), IRD, CNES, 14 av. E. Belin, 31400 Toulouse, France. Key words: Abstract: Shortening of the continental lithosphere is generally accommodated by Andes; the growth of crustal wedges building above megathrusts in the mantle flat-slab subduction; lithosphere. We show that the locus of shortening in the western margin crustal wedge; of South America has largely been controlled by the geometry of the tectonics slab. Numerical models confirm that horizontal subduction favors compression far from the trench, above the asthenospheric wedge and steeply dipping segment of the subducting slab. As a result, a second crustal wedge grows in the hinterland of the continent, and widens the Andes. In the Bolivian orocline, this wedge corresponds to the Eastern Cordillera, whose growth was triggered by a major episode of horizontal subduction. When the slab returned to a steeper dip angle, shortening and uplift pursued, facilitated by the structural and thermo-chemical alteration of the continental lithosphere. We review the successive episodes of horizontal subduction that have occurred beneath South America at different latitudes and show that they explain the diachronic widening of the Andes. -
New Seismological Data from the Calabrian Arc Reveal Arc-Orthogonal
www.nature.com/scientificreports OPEN New seismological data from the Calabrian arc reveal arc‑orthogonal extension across the subduction zone Tiziana Sgroi1*, Alina Polonia2,5, Graziella Barberi3,5, Andrea Billi4,5 & Luca Gasperini2,5 The Calabrian Arc subduction‑rollback system along the convergent Africa/Eurasia plate boundary is among the most active geological structures in the Mediterranean Sea. However, its seismogenic behaviour is largely unknown, mostly due to the lack of seismological observations. We studied low‑to‑moderate magnitude earthquakes recorded by the seismic network onshore, integrated by data from a seafoor observatory (NEMO‑SN1), to compute a lithospheric velocity model for the western Ionian Sea, and relocate seismic events along major tectonic structures. Spatial changes in the depth distribution of earthquakes highlight a major lithospheric boundary constituted by the Ionian Fault, which separates two sectors where thickness of the seismogenic layer varies over 40 km. This regional tectonic boundary represents the eastern limit of a domain characterized by thinner lithosphere, arc‑orthogonal extension, and transtensional tectonic deformation. Occurrence of a few thrust‑type earthquakes in the accretionary wedge may suggest a locked subduction interface in a complex tectonic setting, which involves the interplay between arc‑orthogonal extension and plate convergence. We fnally note that distribution of earthquakes and associated extensional deformation in the Messina Straits region could be explained by right‑lateral displacement along the Ionian Fault. This observation could shed new light on proposed mechanisms for the 1908 Messina earthquake. Te western Ionian Sea and the Eastern Sicily margin (Fig. 1) are actively deforming area 1–5, which encompass diferent geodynamic domains, including Mesozoic rifed margins and the Calabrian Arc subduction system along a geometrically complex Africa/Eurasia plate boundary. -
Regional Variations of the Cutoff Depth of Seismicity in the Crust and Their Relation to Heat Flow and Large Inland-Earthquakes
J. Phys. Earth, 38, 223-250, 1990 Regional Variations of the Cutoff Depth of Seismicity in the Crust and Their Relation to Heat Flow and Large Inland-Earthquakes Kiyoshi Ito * Regional Center for Earthquake Prediction, Faculty of Science, Kyoto University, Takatsuki 569, Japan More than 8,000 earthquakes have been relocated to derive regional variations of the seismic-aseismic boundary in the mid-crust of the northern Kinki district of Japan. The boundary depths are estimated as 13-15 and 18-20 km in the southwestern and northeastern parts of the study area, respectively. The relationship between the seismic cutoff depth and the cause of large intraplate earthquakes is studied, making use of the present observations and other available data of seismicity and surface heat-flow, on the basis of the brittle-ductile transition of rock deformation. The regional variations in the cutoff depth of seismicity appear to be well correlated with the thermal structure of the crust. The cutoff depths in various heat-flow provinces in Japan and other countries are found to be inversely proportional to the surface heat-flow values, with the depths roughly corresponding to isotherms of 200-400•Ž. The shape of the depth-frequency distribution of earthquakes calculated from high-quality data is quite similar to that of the shear resistance calculated using a simple brittle-ductile transition model. Large intraplate earthquakes appear to originate at the peak or just below the peak in the depth-frequency distribution, which also corresponds to the deepest portion of the seismogenic layer. Furthermore, in the source area of large earthquakes, rupture seems to start where sharp changes occur in the cutoff depth of seismicity.