Effective Majorana Mass Matrix from Tau and Pseudoscalar Meson Lepton Number Violating Decays
Total Page:16
File Type:pdf, Size:1020Kb
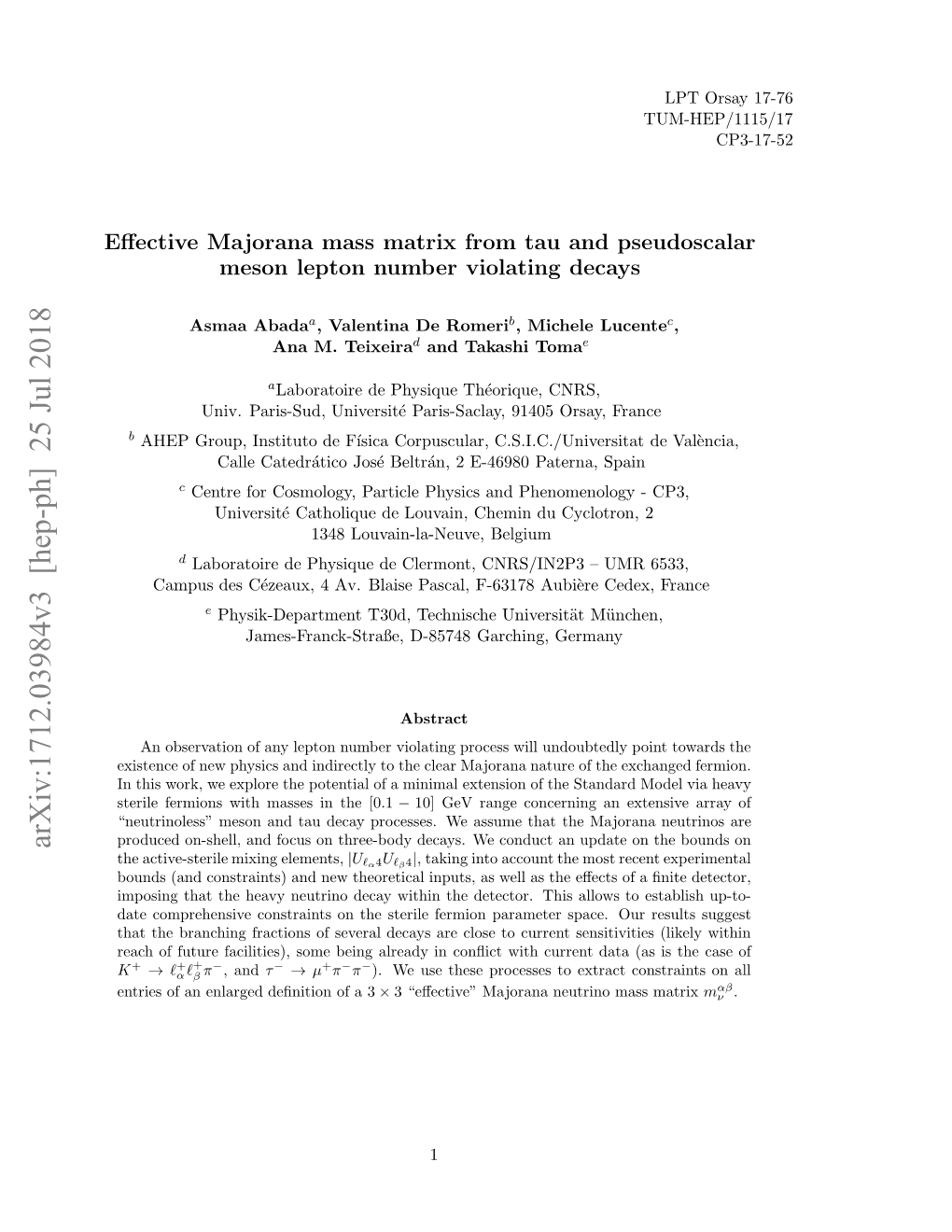
Load more
Recommended publications
-
Reconstruction of Semileptonic K0 Decays at Babar
Reconstruction of Semileptonic K0 Decays at BaBar Henry Hinnefeld 2010 NSF/REU Program Physics Department, University of Notre Dame Advisor: Dr. John LoSecco Abstract The oscillations observed in a pion composed of a superposition of energy states can provide a valuable tool with which to examine recoiling particles produced along with the pion in a two body decay. By characterizing these oscillation in the D+ ! π+ K0 decay we develop a technique that can be applied to other similar decays. The neutral kaons produced in the D+ ! π+ K0 decay are generated in flavor eigenstates due to their production via the weak force. Kaon flavor eigenstates differ from kaon mass eigenstates so the K0 can be equally represented as a superposition of mass 0 0 eigenstates, labelled KS and KL. Conservation of energy and momentum require that the recoiling π also be in an entangled superposition of energy states. The K0 flavor can be determined by 0 measuring the lepton charge in a KL ! π l ν decay. A central difficulty with this method is the 0 accurate reconstruction of KLs in experimental data without the missing information carried off by the (undetected) neutrino. Using data generated at the Stanford Linear Accelerator (SLAC) and software created as part of the BaBar experiment I developed a set of kinematic, geometric, 0 and statistical filters that extract lists of KL candidates from experimental data. The cuts were first developed by examining simulated Monte Carlo data, and were later refined by examining 0 + − 0 trends in data from the KL ! π π π decay. -
Study of Exclusive Charmless Semileptonic Decays of the B Meson
SLAC-R-743 Study of Exclusive Charmless Semileptonic Decays of the B Meson Amanda Jacqueline Weinstein SLAC-Report-743 December 2004 Prepared for the Department of Energy under contract number DE-AC02-76SF00515 This document, and the material and data contained therein, was developed under sponsorship of the United States Government. Neither the United States nor the Department of Energy, nor the Leland Stanford Junior University, nor their employees, nor their respective contractors, subcontractors, or their employees, makes an warranty, express or implied, or assumes any liability of responsibility for accuracy, completeness or useful- ness of any information, apparatus, product or process disclosed, or represents that its use will not infringe privately owned rights. Mention of any product, its manufacturer, or suppliers shall not, nor is it intended to, imply approval, disapproval, or fitness of any particular use. A royalty-free, nonexclusive right to use and dis- seminate same for any purpose whatsoever, is expressly reserved to the United States and the University. STUDY OF EXCLUSIVE CHARMLESS SEMILEPTONIC DECAYS OF THE B MESON a dissertation submitted to the department of physics and the committee on graduate studies of stanford university in partial fulfillment of the requirements for the degree of doctor of philosophy Amanda Jacqueline Weinstein December 2004 c Copyright by Amanda Jacqueline Weinstein 2005 All Rights Reserved ii I certify that I have read this dissertation and that, in my opinion, it is fully adequate in scope and quality as a dissertation for the degree of Doctor of Philosophy. Jonathan Dorfan (Principal Adviser) I certify that I have read this dissertation and that, in my opinion, it is fully adequate in scope and quality as a dissertation for the degree of Doctor of Philosophy. -
Phenomenology of Gev-Scale Heavy Neutral Leptons Arxiv:1805.08567
Prepared for submission to JHEP INR-TH-2018-014 Phenomenology of GeV-scale Heavy Neutral Leptons Kyrylo Bondarenko,1 Alexey Boyarsky,1 Dmitry Gorbunov,2;3 Oleg Ruchayskiy4 1Intituut-Lorentz, Leiden University, Niels Bohrweg 2, 2333 CA Leiden, The Netherlands 2Institute for Nuclear Research of the Russian Academy of Sciences, Moscow 117312, Russia 3Moscow Institute of Physics and Technology, Dolgoprudny 141700, Russia 4Discovery Center, Niels Bohr Institute, Copenhagen University, Blegdamsvej 17, DK- 2100 Copenhagen, Denmark E-mail: [email protected], [email protected], [email protected], [email protected] Abstract: We review and revise phenomenology of the GeV-scale heavy neutral leptons (HNLs). We extend the previous analyses by including more channels of HNLs production and decay and provide with more refined treatment, including QCD corrections for the HNLs of masses (1) GeV. We summarize the relevance O of individual production and decay channels for different masses, resolving a few discrepancies in the literature. Our final results are directly suitable for sensitivity studies of particle physics experiments (ranging from proton beam-dump to the LHC) aiming at searches for heavy neutral leptons. arXiv:1805.08567v3 [hep-ph] 9 Nov 2018 ArXiv ePrint: 1805.08567 Contents 1 Introduction: heavy neutral leptons1 1.1 General introduction to heavy neutral leptons2 2 HNL production in proton fixed target experiments3 2.1 Production from hadrons3 2.1.1 Production from light unflavored and strange mesons5 2.1.2 -
B Meson Decays Marina Artuso1, Elisabetta Barberio2 and Sheldon Stone*1
Review Open Access B meson decays Marina Artuso1, Elisabetta Barberio2 and Sheldon Stone*1 Address: 1Department of Physics, Syracuse University, Syracuse, NY 13244, USA and 2School of Physics, University of Melbourne, Victoria 3010, Australia Email: Marina Artuso - [email protected]; Elisabetta Barberio - [email protected]; Sheldon Stone* - [email protected] * Corresponding author Published: 20 February 2009 Received: 20 February 2009 Accepted: 20 February 2009 PMC Physics A 2009, 3:3 doi:10.1186/1754-0410-3-3 This article is available from: http://www.physmathcentral.com/1754-0410/3/3 © 2009 Stone et al This is an Open Access article distributed under the terms of the Creative Commons Attribution License (http://creativecommons.org/ licenses/by/2.0), which permits unrestricted use, distribution, and reproduction in any medium, provided the original work is properly cited. Abstract We discuss the most important Physics thus far extracted from studies of B meson decays. Measurements of the four CP violating angles accessible in B decay are reviewed as well as direct CP violation. A detailed discussion of the measurements of the CKM elements Vcb and Vub from semileptonic decays is given, and the differences between resulting values using inclusive decays versus exclusive decays is discussed. Measurements of "rare" decays are also reviewed. We point out where CP violating and rare decays could lead to observations of physics beyond that of the Standard Model in future experiments. If such physics is found by directly observation of new particles, e.g. in LHC experiments, B decays can play a decisive role in interpreting the nature of these particles. -
Study of KS Semileptonic Decays and CPT Test with the KLOE Detector
Study of K semileptonic decays and test with S CPT the KLOE detector Daria Kami´nska on behalf of the KLOE-2 Collaboration The Marian Smoluchowski Institute of Physics, Jagiellonian University Lojasiewicza 11, 30-348 Krak´ow,Poland E-mail: [email protected] Abstract. Study of semileptonic decays of neutral kaons allows to perform a test of discrete symmetries, as well as basic principles of the Standard Model. In this paper a general review on dependency between charge asymmetry constructed for semileptonic decays of short- and long-lived kaons and CPT symmetry is given. The current status of determination of charge 5 asymmetry for short-lived kaon, obtained by reconstruction of about 10 KS ! πeν decays collected at DAΦNE with the KLOE detector is also reviewed. 1. Introduction Discrete symmetries of nature such as charge conjugation ( ), parity ( ) or time reversal ( ) do not lead to new conserved quantities. Nevertheless, all ofC the mentionedP symmetries playsT an important role in particle physics, especially in calculations of the cross sections and decay rates. Weak interaction does not conserve the , , or combined symmetry. However, up to now, there is no indication of symmetryC P violationT [1], whichCP would also imply the break of Lorentz symmetry [2]. A specialCPT role in violation searches plays a neutral kaon system which, due to a sensitivity to a variety of symmetryCPT violation effects, is one of the best candidates for such kind of studies. One of the possible tests is based on comparison between semileptonic asymmetry in KS decays (AS) and the analogous asymmetry in KL decays (AL). -
B Decays and CP Violation
CERN-TH/96-55 hep-ph/9604412 B Decays and CP Violation Matthias Neubert Theory Division, CERN, CH-1211 Geneva 23, Switzerland Abstract We review the status of the theory and phenomenology of heavy-quark symmetry, exclusive weak decays of B mesons, inclusive decay rates and life- times of b hadrons, and CP violation in B-meson decays. (To appear in International Journal of Modern Physics A) CERN-TH/96-55 April 1996 International Journal of Modern Physics A, cf World Scientific Publishing Company B DECAYS AND CP VIOLATION MATTHIAS NEUBERT Theory Division, CERN CH-1211 Geneva 23, Switzerland Received (received date) Revised (revised date) We review the status of the theory and phenomenology of heavy-quark symmetry, ex- clusive weak decays of B mesons, inclusive decay rates and lifetimes of b hadrons, and CP violation in B-meson decays. 1. Introduction The rich phenomenology of weak decays has always been a source of information about the nature of elementary particle interactions. A long time ago, β-and µ-decay experiments revealed the structure of the effective flavour-changing inter- actions at low momentum transfer. Today, weak decays of hadrons containing heavy quarks are employed for tests of the Standard Model and measurements of its parameters. In particular, they offer the most direct way to determine the weak mixing angles, to test the unitarity of the Cabibbo–Kobayashi–Maskawa (CKM) matrix, and to explore the physics of CP violation. On the other hand, hadronic weak decays also serve as a probe of that part of strong-interaction phenomenology which is least understood: the confinement of quarks and gluons inside hadrons. -
Weak Interactioninteraction
WeakWeak InteractionInteraction OutlineOutline Introduction to weak interactions Charged current (CC) interactions Neutral current (NC) Weak vector bosons W± and Z0 Weak charged interactions Beta decay Flavour changing charged current W± boson propagator Fermi coupling constant Parity violation Muon decay Decay rate /lifetime Lepton Universality W± boson couplings for leptons Tau decays Weak quark decays W± boson couplings for quarks Cabibbo angle, CKM mechanism Spectator model Nuclear and Particle Physics Franz Muheim 1 IntroductionIntroduction Weak Interactions Account for large variety of physical processes Muon and Tau decays, Neutrino interactions Decays of lightest mesons and baryons Z0 and W± boson production at √s ~ O(100 GeV) Natural radioactivity, fission, fusion (sun) Major Characteristics Long lifetimes Small cross sections (not always) “Quantum Flavour Dynamics” Charged Current (CC) Neutral Current (NC) mediated by exchange of W± boson Z0 boson Intermediate vector bosons MW = 80.4 GeV ± 0 W and Z have mass MZ = 91.2 GeV Self Interactions of W± and Z0 also W± and γ Nuclear and Particle Physics Franz Muheim 2 BetaBeta DecayDecay Weak Nuclear Decays See also Nuclear Physics Recall β+ = e+ β- = e- Continuous energy spectrum of e- or e+ Î 3-body decay, Pauli postulates neutrino, 1930 Interpretation Fermi, 1932 Bound n or p decay − n → pe ν e ⎛ − t ⎞ N(t) = N(0)exp⎜ ⎟ ⎝ τ n ⎠ p → ne +ν (bound p) τ n = 885.7 ± 0.8 s e 32 τ 1 = τ n ln 2 = 613.9 ± 0.6 s τ p > 10 y (p stable) 2 Modern quark level picture Weak charged current mediated -
Search for New Mechanism of CP Violation Through Tau Decay and Semilpetonic Decay of Hadrons *
SLAC-PUB-7360 November 1996 Search For New Mechanism of CP Violation through Tau Decay and Semilpetonic Decay of Hadrons * Yung Su Tsai Stanford Linear Accelerator Center Stanford University, Stanford, California 94309 e-mail:[email protected] Abstract If CP is violated in any decay process involving leptons it will signify the existense of a new force (called the X boson) responsible for CP violation that may be the key to understanding matter-antimatter asymmetry in the universe. We discuss the r _ signatures of CP violation in (1) the decay of tau lepton, and (2) the semileptonic decay of K, Ii’, D, B and t particles by measuring the polarization of the charged lepton in the decay. We discuss how the coupling constants and their phases of the coupling of the X boson to 9 quark vertices and 3 lepton vertices can be obtained through 12 decay processes. Presented to the Proceedings of the Fourth International Workshop on Tau Physics Estes Park, Colorado 16-19 September 1996 c - *Work supported by the Department of Energy, contract DE-AC03-76SF00515. 1 INTRODUCTION The only particle that exhibits CP violation so far is the neutral li’ [l] and in a few years we would know whether B and D mesons will have CP violation from the B factories. The standard theory of CP violation due to Kobayashi and Maskawa predicts [2, 31 that th ere is no CP violation whenever lepton is involved in the decay either as a parent or as a daughter. This prediction applies for example to the decay of a muon and a tau lepton or semileptonic decay of a hadron such as 7r, Ii’, D, B or t as shown in Table 1. -
Heavy-Quark Physics and Cp Violation
COURSE HEAVYQUARK PHYSICS AND CP VIOLATION Jerey D Richman University of California y Santa Barbara California USA y Email richmancharmphysicsucsbedu c Elsevier Science BV Al l rights reserved Photograph of Lecturer Contents Intro duction Roadmap and Overview of Bottom and Charm Physics Intro duction to the Cabibb oKobayashiMaskawa Matrix and a First Lo ok at CP Violation Exp erimental Challenges and Approaches in HeavyQuark Physics Historical Persp ective Bumps in the Road and Lessons in Data Analysis Avery short history of heavyquark physics Bumps in the road case studies Some rules for data analysis Leptonic Decays Intro duction to leptonic decays Measurements of leptonic decays Lattice calculations of leptonic decay constants Semileptonic Decays Intro duction to semileptonic decays Dynamics of semileptonic decay Heavy quark eective theory and semileptonic decays Inclusive semileptonic decay and jV j cb Leptonendp oint region in semileptonic B deca y and jV j ub Form factors and kinematic distributions for exclusive semileptonic decay HQET predictions and the IsgurWise function Exclusive semileptonic decay jV j and jV j cb ub Hadronic Decays Lifetimes and Rare Decays Hadronic Decays Lifetimes Rare decays CP Violation and Oscillations Intro duction to CP violation CP violation and cosmology CP violation in decay direct CP violation CP violation in mixing indirect CP violation Phenomenology of mixing CP violation due to interference b etween mixing and decay Acknowledgements -
Search for New Mechanism of CP Violation Through Decay of Leptons and Semileptonic Decay of Hadrons
SLAC{PUB{7482 Novemb er 1996 SearchFor New Mechanism of CP Violation through Decay of Leptons and Semileptonic Decay of Hadrons Yung Su Tsai Stanford Linear Accelerator Center Stanford University, Stanford, California 94309 e-mail:[email protected] Abstract If CP is violated in any decay pro cess involving leptons it will signify the existense of a new scalar b oson called the X b oson resp onsible for CP violation that maybe the key to understanding matter-antimatter asymmetry in the universe. We discuss the signatures of CP violation in 1 the decay of tau and mu leptons, and 2 the semileptonic decayof ,K, D, B and t particles by measuring the p olarization of the charged lepton in the decay.We discuss how the coupling constants and their phases of the coupling of the X b oson to 9 quark vertices and 3 lepton vertices can b e obtained through 12 decay pro cesses. Presented to the Pro ceedings of the Physics Symp osium on the 50th Anniversary of the Taiwan University Taip ei, Taiwan 28-30 Decemb er 1996 Work supp orted by the Department of Energy, contract DE{AC03{76SF00515. 1 INTRODUCTION The only particle that exhibits CP violation so far is the neutral K [1] and in a few years wewould know whether B and D mesons will have CP violation from the B factories. The standard theory of CP violation due to Kobayashi and Maskawa predicts [2, 3] that there is no CP violation whenever lepton is involved in the decay either as a parent or as a daughter. -
Comments on CP, T and CPT Violation in Neutral Kaon Decays
CERN-TH/99-60 OUTP-99-17P hep-ph/9903386 Comments on CP, T and CPT Violation in Neutral Kaon Decays John Ellisa and N.E. Mavromatosa;b a CERN, Theory Division, CH-1211 Geneva 23, Switzerland. b University of Oxford, Department of Physics, Theoretical Physics, 1 Keble Road, Oxford OX1 3NP, U.K. Contribution to the Festschrift for L.B. Okun, to appear in a special issue of Physics Reports eds. V.L. Telegdi and K. Winter Abstract We comment on CP, T and CPT violation in the light of interesting new data from the CPLEAR and KTeV Collaborations on neutral kaon decay asymmetries. Other recent data from the CPLEAR experiment, constraining possible violations of CPT and the ∆S =∆Qrule, exclude the possibility that the semileptonic-decay asymmetry AT measured by CPLEAR could be solely due to CPT violation, confirming that their data constitute direct evidence for T violation. The CP-violating asymmetry in K e e+π π+ L → − − recently measured by the KTeV Collaboration does not by itself provide direct evidence for T violation, but we use it to place new bounds on CPT violation. 1 Introduction 0 Ever since the discovery of CP violation in KL 2π decay by Christenson, Cronin, Fitch and Turlay in 1964 [1], its understanding has→ been a high experimental and theoretical priority. Until recently, mixing in the K0 K0 mass matrix was the only known source of CP violation, since it was sufficient− by itself to explain 0 the observations of CP violation in other KS;L decays, no CP violation was seen in experiments on K±,charmorB-meson decays, and searches for electric dipole moments only gave upper limits [2]. -
Arxiv:Hep-Ph/9508389V1 28 Aug 1995 Metry, Auso H H W Ope Mltd Aisfrtedcy of Decays the for Are Ratios There Amplitude Here Complex Two System
CP VIOLATION: A THEORETICAL REVIEW ∗ R. D. Peccei Department of Physics, University of California at Los Angeles, Los Angeles, CA 90095-1547 Abstract This review of CP violation focuses on the status of the subject and its likely future development through experiments in the Kaon system and with B-decays. Although present observations of CP violation are perfectly consistent with the CKM model, we discuss the theoretical and experimental difficulties which must be faced to establish this conclusively. In so doing, theoretical predictions and experimental prospects for detecting ∆S = 1 CP violation through measurements of ǫ′/ǫ and of rare K decays are reviewed. The crucial role that B CP-violating experiments will play in elucidating this issue is emphasized . The importance of looking for evidence for non-CKM CP-violating phases, through a search for a non-vanishing transverse muon polarization in Kµ3 decays, is also stressed. 1 Introduction The discovery more than 30 years ago of the decay KL 2π by Christianson, Cronin, Fitch and Turlay 1) provided the first indication that CP, like parity,→ was also not a good symmetry of nature. It is rather surprising that, for such a mature subject, we have still so little experimental arXiv:hep-ph/9508389v1 28 Aug 1995 information available. Indeed, the only firm evidence for CP violation to date remains that deduced from meausurements in the neutral Kaon system. Here there are five parameters meausured: the + − values of the the two complex amplitude ratios for the decays of KL and KS to π π ′ o o ′ (n+− = ǫ + ǫ ) and to π π (ηoo = ǫ 2ǫ ), plus the semileptonic asymmetry in KL decays (AKL ) 1.