Adaptations in Allopatric Populations of Triakis Megalopterus Isolated by the Benguela Current
Total Page:16
File Type:pdf, Size:1020Kb
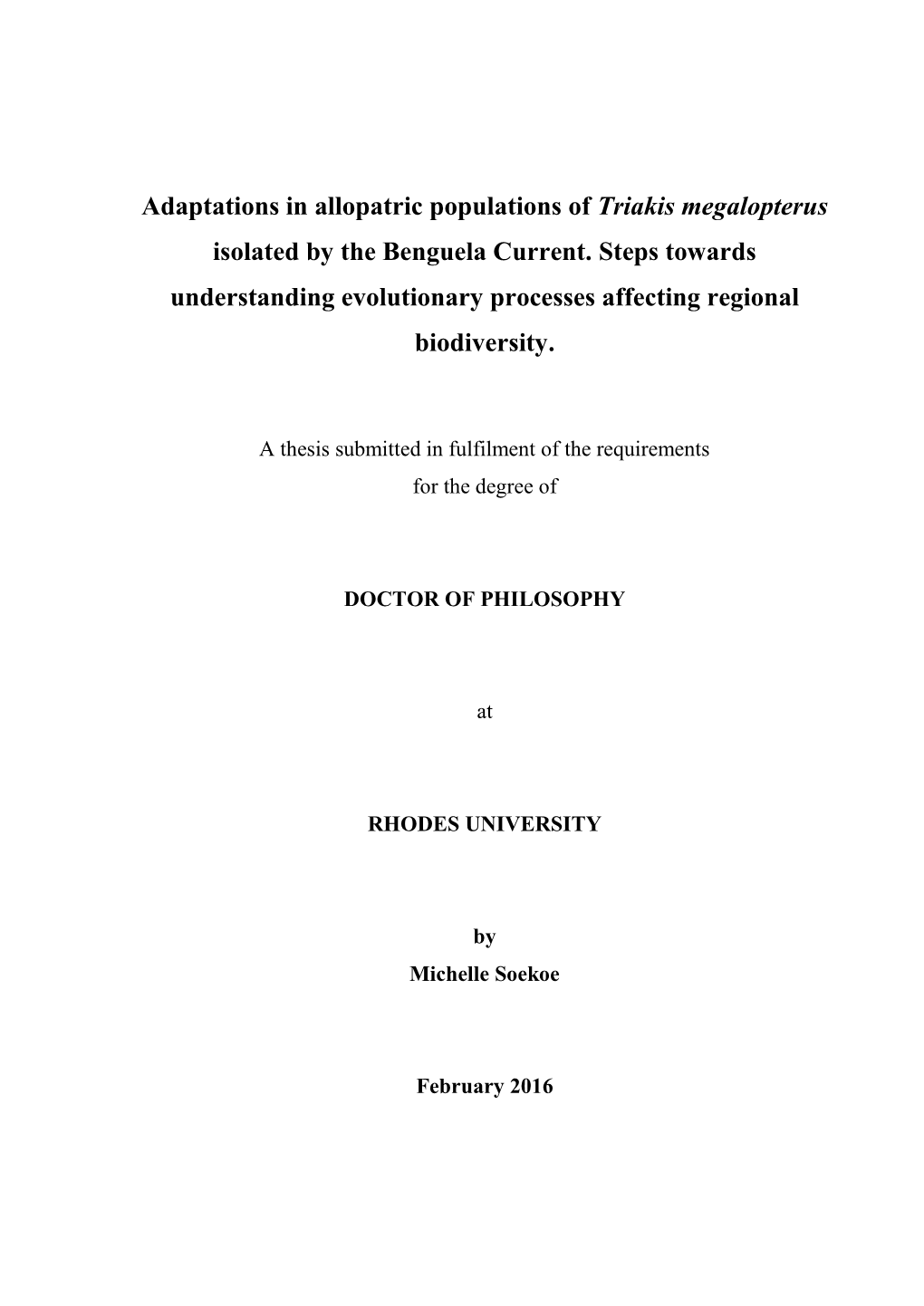
Load more
Recommended publications
-
§4-71-6.5 LIST of CONDITIONALLY APPROVED ANIMALS November
§4-71-6.5 LIST OF CONDITIONALLY APPROVED ANIMALS November 28, 2006 SCIENTIFIC NAME COMMON NAME INVERTEBRATES PHYLUM Annelida CLASS Oligochaeta ORDER Plesiopora FAMILY Tubificidae Tubifex (all species in genus) worm, tubifex PHYLUM Arthropoda CLASS Crustacea ORDER Anostraca FAMILY Artemiidae Artemia (all species in genus) shrimp, brine ORDER Cladocera FAMILY Daphnidae Daphnia (all species in genus) flea, water ORDER Decapoda FAMILY Atelecyclidae Erimacrus isenbeckii crab, horsehair FAMILY Cancridae Cancer antennarius crab, California rock Cancer anthonyi crab, yellowstone Cancer borealis crab, Jonah Cancer magister crab, dungeness Cancer productus crab, rock (red) FAMILY Geryonidae Geryon affinis crab, golden FAMILY Lithodidae Paralithodes camtschatica crab, Alaskan king FAMILY Majidae Chionocetes bairdi crab, snow Chionocetes opilio crab, snow 1 CONDITIONAL ANIMAL LIST §4-71-6.5 SCIENTIFIC NAME COMMON NAME Chionocetes tanneri crab, snow FAMILY Nephropidae Homarus (all species in genus) lobster, true FAMILY Palaemonidae Macrobrachium lar shrimp, freshwater Macrobrachium rosenbergi prawn, giant long-legged FAMILY Palinuridae Jasus (all species in genus) crayfish, saltwater; lobster Panulirus argus lobster, Atlantic spiny Panulirus longipes femoristriga crayfish, saltwater Panulirus pencillatus lobster, spiny FAMILY Portunidae Callinectes sapidus crab, blue Scylla serrata crab, Samoan; serrate, swimming FAMILY Raninidae Ranina ranina crab, spanner; red frog, Hawaiian CLASS Insecta ORDER Coleoptera FAMILY Tenebrionidae Tenebrio molitor mealworm, -
Sharks for the Aquarium and Considerations for Their Selection1 Alexis L
FA179 Sharks for the Aquarium and Considerations for Their Selection1 Alexis L. Morris, Elisa J. Livengood, and Frank A. Chapman2 Introduction The Lore of the Shark Sharks are magnificent animals and an exciting group Though it has been some 35 years since the shark in Steven of fishes. As a group, sharks, rays, and skates belong to Spielberg’s Jaws bit into its first unsuspecting ocean swim- the biological taxonomic class called Chondrichthyes, or mer and despite the fact that the risk of shark-bite is very cartilaginous fishes (elasmobranchs). The entire supporting small, fear of sharks still makes some people afraid to swim structure of these fish is composed primarily of cartilage in the ocean. (The chance of being struck by lightning is rather than bone. There are some 400 described species of greater than the chance of shark attack.) The most en- sharks, which come in all different sizes from the 40-foot- grained shark image that comes to a person’s mind is a giant long whale shark (Rhincodon typus) to the 2-foot-long conical snout lined with multiple rows of teeth efficient at marble catshark (Atelomycterus macleayi). tearing, chomping, or crushing prey, and those lifeless and staring eyes. The very adaptations that make sharks such Although sharks have been kept in public aquariums successful predators also make some people unnecessarily since the 1860s, advances in marine aquarium systems frightened of them. This is unfortunate, since sharks are technology and increased understanding of shark biology interesting creatures and much more than ill-perceived and husbandry now allow hobbyists to maintain and enjoy mindless eating machines. -
Korea, Republic Of
Ministry for Food, Agriculture, Forestry and Fisheries National Plan of Action for the Conservation and Management of Sharks The Republic of Korea August, 2011 MIFAFF Ministry for Food, Agriculture, Forestry and Fisheries I. Introduction ············································································ 2 II. Current State of Shark Management in Korea ························· 3 2.1. Spatial Distribution of Sharks and Related Fisheries ····················· 3 2.2. Shark Catch Statistics ··································································· 7 2.3. Domestic and International Trade of Sharks ································· 8 2.4. Management of Sharks ································································· 9 III. National Plan of Action for the Conservation and Management of Sharks · 10 3.1. Objectives and Scope of National Plan of Action for Sharks······· 10 3.2. Data Collection, Analysis and Assessment ··································· 11 3.3. Conservation and Management Measures ···································· 13 3.4. Monitoring, Control and Surveillance ··········································· 15 3.5. Maximizing the Utilization of Sharks Caught ······························· 16 3.6. Research and Development ························································· 17 3.7. Public Information and Promotion ··············································· 18 3.8. International Cooperation ····························································· 19 3.9. Other Issues ················································································ -
An Investigation on Fishes of Bandirma Bay (Sea of Marmara)
BAÜ Fen Bil. Enst. Dergisi (2004).6.2 AN INVESTIGATION ON FISHES OF BANDIRMA BAY (SEA OF MARMARA) Hatice TORCU KOÇ University of Balikesir, Faculty of Science and Arts, Department of Hydrobiology, 10100, Balikesir, Turkey ABSTRACT This investigation was carried out for the determination of fish species living in Bandırma Bay (Sea of Marmara). Morphometric and meristic characters of of fishes caught by trawl and various nets in Bandırma Bay in the years of 1998-1999 were examined and some morphological, ecological properties, and local names of 34 determined species are given. Key Words: Fish Species, Systematic, Bandırma Bay BANDIRMA KÖRFEZİ (MARMARA DENİZİ) BALIKLARI ÜZERİNE BİR ARAŞTIRMA ÖZET Bu araştırma Bandırma Körfezi (Marmara Denizi)’nde yaşayan balık türlerini belirlemek amacıyla yapılmıştır. 1998-1999 yılları arasında körfez içinde trol ve çeşitli ağlar ile yakalanan balıkların morfometrik ve meristik karakterleri incelenmiş ve saptanan 34 türün bazı morfolojik, ekolojik özellikleri, ve yerel isimleri verilmiştir. Anahtar Kelimeler: Balık türleri, Sistematik, Bandırma Körfezi 1. INTRODUCTION Research on the sea fauna along the coasts of Turkey was initiated by foreign researchers at the begining of the 20th century and entered an intensive stage with Turkish researchers in the 1940s. However, the fish fauna of Turkish seas has still not been fully determined. Of these researchers, Tortonese (1) listed 300 species. Papaconstantinou and Tsimenids (2) listed 33 species. Papaconstantinou (3) listed the most of 447 species for Aegean Sea. Slastenenko (4) listed 200 species for Sea of Marmara and 189 species for Black Sea. Tortonese (1) reported 540 fish species in whole of Mediterranean. Demetropoulos and Neocleous (5) gave a list of fishes for Cyprus area. -
ENVIRONMENTAL STUDIES Vol
PURARI RIVER (WABO) HYDROELECTRIC SCHEME ENVIRONMENTAL STUDIES Vol. 3 THE ECOLOGICA SIGNIFICANCE AND ECONOMIC IMPORTANCE OF THE MANGROVE ~AND ESTU.A.INE COMMUNITIES OF THE GULF PROVINCE, PAPUA NEW GUINEA Aby David S.Liem and Allan K. Haines .:N. IA 1-:" .": ". ' A, _"Gulf of Papua \-.. , . .. Office of Environment and Gonservatior, Central Government Offices, Waigani, Department o Minerals and Energy,and P.O. Box 2352, Kot.edobu !" " ' "car ' - ;' , ,-9"... 1977 "~ ~ u l -&,dJ&.3.,' -a,7- ..g=.<"- " - Papua New Guinea -.4- "-4-4 , ' -'., O~Cx c.A -6 Editor: Dr. T. Petr, Office of Environment and Conservation, Central Government Offices, Waigani, Papua New Guinea Authors: David S. Liem, Wildlife Division, Department of Natural Resources, Port Moresby, Papua New Guinea Allan K. Haines, Fisher;es Division, Department of Primary Industry, Konedobu, Papua New Guinea Reports p--blished in the series -ur'ri River (.:abo) Hyrcelectr.c S-hane: Environmental Studies Vol.l: Workshop 6 May 1977 (Ed.by T.Petr) (1977) Vol.2: Computer simulaticn of the impact of the Wabo hydroelectric scheme on the sediment balance of the Lower Purari (by G.Pickup) (1977) Vol.3: The ecological significance and economic importance of the mangrove and estuarine communities of the Gulf Province,Papua New Guinea (by D.S.Liem & A.K.Haines) (1977) Vol.4: The pawaia of the Upper Purari (Gulf Province,Papua New Guinea) (by C.Warrillow)( 1978) Vol.5: An archaeological and ethnographic survey of the Purari River (Wabo) dam site and reservoir (by S.J.Egloff & R.Kaiku) (1978) In -
Morphometric and Meristic Variation in Two
Simon et al. / J Zhejiang Univ-Sci B (Biomed & Biotechnol) 2010 11(11):871-879 871 Journal of Zhejiang University-SCIENCE B (Biomedicine & Biotechnology) ISSN 1673-1581 (Print); ISSN 1862-1783 (Online) www.zju.edu.cn/jzus; www.springerlink.com E-mail: [email protected] Morphometric and meristic variation in two congeneric archer fishes Toxotes chatareus (Hamilton 1822) and Toxotes jaculatrix * (Pallas 1767) inhabiting Malaysian coastal waters K. D. SIMON†1, Y. BAKAR2, S. E. TEMPLE3, A. G. MAZLAN†‡4 (1Marine Science Programme, School of Environmental and Natural Resource Sciences, Faculty of Science and Technology, Universiti Kebangsaan Malaysia, 43600 UKM Bangi, Selangor D.E., Malaysia) (2Biology Programme, School of Biological Sciences, Universiti Kebangsaan Malaysia, 43600 UKM Bangi, Selangor D.E., Malaysia) (3School of Biomedical Sciences, University of Queensland, Brisbane, Queensland 4072, Australia) (4Marine Ecosystem Research Centre, Faculty of Science and Technology, Universiti Kebangsaan Malaysia, 43600 UKM Bangi, Selangor D.E., Malaysia) †E-mail: [email protected]; [email protected] Received Feb. 18, 2010; Revision accepted Aug. 3, 2010; Crosschecked Oct. 8, 2010 Abstract: A simple yet useful criterion based on external markings and/or number of dorsal spines is currently used to differentiate two congeneric archer fish species Toxotes chatareus and Toxotes jaculatrix. Here we investigate other morphometric and meristic characters that can also be used to differentiate these two species. Principal component and/or discriminant functions revealed that meristic characters were highly correlated with pectoral fin ray count, number of lateral line scales, as well as number of anal fin rays. The results indicate that T. chatareus can be distin- guished from T. -
DEEP SEA LEBANON RESULTS of the 2016 EXPEDITION EXPLORING SUBMARINE CANYONS Towards Deep-Sea Conservation in Lebanon Project
DEEP SEA LEBANON RESULTS OF THE 2016 EXPEDITION EXPLORING SUBMARINE CANYONS Towards Deep-Sea Conservation in Lebanon Project March 2018 DEEP SEA LEBANON RESULTS OF THE 2016 EXPEDITION EXPLORING SUBMARINE CANYONS Towards Deep-Sea Conservation in Lebanon Project Citation: Aguilar, R., García, S., Perry, A.L., Alvarez, H., Blanco, J., Bitar, G. 2018. 2016 Deep-sea Lebanon Expedition: Exploring Submarine Canyons. Oceana, Madrid. 94 p. DOI: 10.31230/osf.io/34cb9 Based on an official request from Lebanon’s Ministry of Environment back in 2013, Oceana has planned and carried out an expedition to survey Lebanese deep-sea canyons and escarpments. Cover: Cerianthus membranaceus © OCEANA All photos are © OCEANA Index 06 Introduction 11 Methods 16 Results 44 Areas 12 Rov surveys 16 Habitat types 44 Tarablus/Batroun 14 Infaunal surveys 16 Coralligenous habitat 44 Jounieh 14 Oceanographic and rhodolith/maërl 45 St. George beds measurements 46 Beirut 19 Sandy bottoms 15 Data analyses 46 Sayniq 15 Collaborations 20 Sandy-muddy bottoms 20 Rocky bottoms 22 Canyon heads 22 Bathyal muds 24 Species 27 Fishes 29 Crustaceans 30 Echinoderms 31 Cnidarians 36 Sponges 38 Molluscs 40 Bryozoans 40 Brachiopods 42 Tunicates 42 Annelids 42 Foraminifera 42 Algae | Deep sea Lebanon OCEANA 47 Human 50 Discussion and 68 Annex 1 85 Annex 2 impacts conclusions 68 Table A1. List of 85 Methodology for 47 Marine litter 51 Main expedition species identified assesing relative 49 Fisheries findings 84 Table A2. List conservation interest of 49 Other observations 52 Key community of threatened types and their species identified survey areas ecological importanc 84 Figure A1. -
Updated Checklist of Marine Fishes (Chordata: Craniata) from Portugal and the Proposed Extension of the Portuguese Continental Shelf
European Journal of Taxonomy 73: 1-73 ISSN 2118-9773 http://dx.doi.org/10.5852/ejt.2014.73 www.europeanjournaloftaxonomy.eu 2014 · Carneiro M. et al. This work is licensed under a Creative Commons Attribution 3.0 License. Monograph urn:lsid:zoobank.org:pub:9A5F217D-8E7B-448A-9CAB-2CCC9CC6F857 Updated checklist of marine fishes (Chordata: Craniata) from Portugal and the proposed extension of the Portuguese continental shelf Miguel CARNEIRO1,5, Rogélia MARTINS2,6, Monica LANDI*,3,7 & Filipe O. COSTA4,8 1,2 DIV-RP (Modelling and Management Fishery Resources Division), Instituto Português do Mar e da Atmosfera, Av. Brasilia 1449-006 Lisboa, Portugal. E-mail: [email protected], [email protected] 3,4 CBMA (Centre of Molecular and Environmental Biology), Department of Biology, University of Minho, Campus de Gualtar, 4710-057 Braga, Portugal. E-mail: [email protected], [email protected] * corresponding author: [email protected] 5 urn:lsid:zoobank.org:author:90A98A50-327E-4648-9DCE-75709C7A2472 6 urn:lsid:zoobank.org:author:1EB6DE00-9E91-407C-B7C4-34F31F29FD88 7 urn:lsid:zoobank.org:author:6D3AC760-77F2-4CFA-B5C7-665CB07F4CEB 8 urn:lsid:zoobank.org:author:48E53CF3-71C8-403C-BECD-10B20B3C15B4 Abstract. The study of the Portuguese marine ichthyofauna has a long historical tradition, rooted back in the 18th Century. Here we present an annotated checklist of the marine fishes from Portuguese waters, including the area encompassed by the proposed extension of the Portuguese continental shelf and the Economic Exclusive Zone (EEZ). The list is based on historical literature records and taxon occurrence data obtained from natural history collections, together with new revisions and occurrences. -
Across-Shelf Larval, Postlarval, and Juvenile Fish Collected at Offshore Oil and Gas Platforms and a Coastal Rock Jetty West of the Mississippi River Delta
OCS Study MMS 2001-077 Coastal Marine Institute Across-Shelf Larval, Postlarval, and Juvenile Fish Collected at Offshore Oil and Gas Platforms and a Coastal Rock Jetty West of the Mississippi River Delta U .S . Department of the Interior AnK Cooperative Agreement Minerals 11Aanagement Service Coastal Marine Institute Adw Gulf of Mexico OCS Region Louisiana State University IR OCS Study MMS 2001-077 Coastal Marine Institute Across-Shelf Larval, Postlarval, and Juvenile Fish Collected at Offshore Oil and Gas Platforms and a Coastal Rock Jetty West of the Mississippi River Delta Authors Frank J. Hernandez, Jr. Richard F. Shaw Joseph S . Cope James G . Ditty Mark C. Benfield Talat Farooqi September 2001 Prepared under MMS Contract 14-35-0001-30660-19926 by Coastal Fisheries Institute Louisiana State University Baton Rouge, Louisiana 70803 Published by U .S. Department of the Interior Cooperative Agreement Minerals Management Service Coastal Marine Institute Gulf of Mexico OCS Region Louisiana State University DISCLAIMER This report was prepared under contract between the Minerals Management Service (MMS) and the Coastal Fisheries Institute (CFI), Louisiana State University (LSU). This report has been technically reviewed by the MMS and it has been approved for publication. Approval does not signify that the contents necessarily reflect the views and policies of LSU or the MMS, nor does mention of trades names or commercial products constitute endorsement or recommendation for use. It is, however, exempt from review and compliance with the MMS editorial standard. REPORT AVAILABILITY Extra copies of the report may be obtained from the Public Information Office (Mail Stop 5034) at the following address : U.S . -
SUPPLEMENTARY ONLINE MATERIAL for New Specimen of the Rare Requiem Shark Eogaleus Bolcensis from the Bolca Lagerstätte, Italy G
http://app.pan.pl/SOM/app65-LaroccaConte_etal_SOM.pdf SUPPLEMENTARY ONLINE MATERIAL FOR New specimen of the rare requiem shark Eogaleus bolcensis from the Bolca Lagerstätte, Italy Gabriele Larocca Conte, Enrico Trevisani, Paolo Guaschi, and Federico Fanti Published in Acta Palaeontologica Polonica 2020 65 (3): 547-560. https://doi.org/10.4202/app.00725.2020 Supplementary Online Material SOM 1. Table 1. Measurements of Galeorhinus cuvieri and Eogaleus bolcensis. Table 2. Age estimates of Bolca specimens according to growth parameters of different extant populations of carcharhiniforms. SOM 2. Measurements of preserved teeth of MSNPV 24625 available at http://app.pan.pl/SOM/app65-LaroccaConte_etal_SOM/SOM_2.xlsx SOM 3. Counts and antero-posterior length of centra of Bolca carcharhiniforms assemblage available at http://app.pan.pl/SOM/app65-LaroccaConte_etal_SOM/SOM_3.xlsx References SOM 1. Table 1. Measurements (in mm) of Galeorhinus cuvieri and Eogaleus bolcensis. %TL = (X/TL) * 100; where %TL, percentage of the total length; X, length of the body segment. ID, morphometric measurement (see Fig. 1A for explanations). “+x” refers to the missing body fragment of the incomplete specimens. Galeorhinus cuvieri Eogaleus bolcensis MGP-PD 8869 C- ID MGP-PD 8871-8872 MCSNV T1124 MCSNV VIIB96-VIIB97 MGGC 1976 MNHN FBol516 MCSNV T311 8870 C cm %TL cm %TL cm %TL cm %TL cm %TL cm %TL cm %TL 1 69.4 1 92 1 83+x - 92 1 67+x - 135 1 - - 2 13.9 20.03 16 17.39 18 - 14.6 15.89 15.5+x - 23 17.04 - - 3 35.5 51.15 46 50 42 - 48.3 52.48 37 - 73 54.07 85.7 - 4 -
Download the Full Article As Pdf ⬇︎
LocalLockdown Diving — Dives Found in Contributors' Backyards Text and photos by Andrey Bizyukin, Larry Cohen, Brent Durand, Dmitry Efremychev, Jennifer Idol, Kate Jonker, Matthew Meier, Pete Mesley, Don Silcock, Olga Torrey and Martin Voeller As many divers face travel restrictions during the coronavi- rus pandemic, our contributors highlight the often overlooked or unsung yet intriguing div- ing that can be found in one's own backyard. X-Ray Mag contributors share their favorite local haunts—from a spring-fed Texan lake to a quarry and a sinkhole in Russia to the tem- perate waters off New Zealand, Japan, South Africa, New Jersey and Northern California to the subtropical waters of Southern California and Sydney, Australia—where they captured compelling underwater images. 58 X-RAY MAG : 101 : 2020 EDITORIAL FEATURES TRAVEL NEWS WRECKS EQUIPMENT BOOKS SCIENCE & ECOLOGY TECH EDUCATION PROFILES PHOTO & VIDEO PORTFOLIO MATTHEW MEIER feature Local Dives School of opaleye and garibaldi among sea grass and feather boa kelp. PREVIOUS PAGE: School of juvenile senorita fish in the kelp and sea grass beds Bat Ray Cove, San Clemente Island, Soupfin (tope) sharks can be seen swimming in California, USA the shallows and among the giant kelp, along with schools of blacksmith and jack mackerels. Text and photos by Matthew Meier California sea lions will swoop through intermittently, and the occasional harbor seal Thankfully, local diving is still possible during will play peak-a-boo in the kelp. the pandemic, and while this dive site requires Under the boat is a sandy bottom where boat access, it is still one of my favorites. -
Investigation Report of Fish and Shellfish Sampled in the Ocean Area Within 20Km Radius of Fukushima Daiichi NPS* (Sampling Period: January – March, 2014)
Investigation Report of Fish and Shellfish Sampled in the Ocean Area Within 20km Radius of Fukushima Daiichi NPS* (Sampling period: January – March, 2014) Tokyo Electric Power Company June 13, 2014 * Exclude the data obtained in the port of Fukushima Daiichi NPS 1. Purpose of the Investigation of Fish and Shellfish Sampled in the Ocean Area Within 20km Radius of Fukushima Daiichi NPS (1) To understand radioactive cesium density by fish species - Comparison with the food standard value (total cesium amount: 100Bq/kg) (2) To understand the geographical distribution of radioactive cesium density of fish and shellfish - Sampling at fixed measurement points (gill net fishing, trawl net fishing) (3) To understand the change of radioactive cesium density of fish and shellfish over time - Accumulating basic data in order to forecast trends 1 2-1. Investigation Results (Radioactive Cesium Density by Fish Species) Approx. 90% of all the measurement results were below the standard value. Standard value: 100 Bq/kg of total amount of radioactive cesium Sampling period: January to March , 2014 Sampling period: October to November , 2013 [Top 3 Density Levels] [Top 3 Density Levels] Number of fish 32 (Unit: Bq/kg (Raw)) 39 (Unit: Bq/kg (Raw)) species (cesium 1. Common skete (cesium exceeding 1. Banded houndshark exceeding the 2. Schlegel's black rockfish the 2. Schlegel's black rockfish standard value: 3. Sebastes cheni standard value: 9) 3. Marbled sole ) 6 [Samples below the [Samples below the detection limit] detection limit] 1. Blue crab 1. Blue crab Number of 241 2. Yellow goosefish 271 2. Roundnose flounder measurements (cesium 3.