2021.01.12.426377V1.Full.Pdf
Total Page:16
File Type:pdf, Size:1020Kb
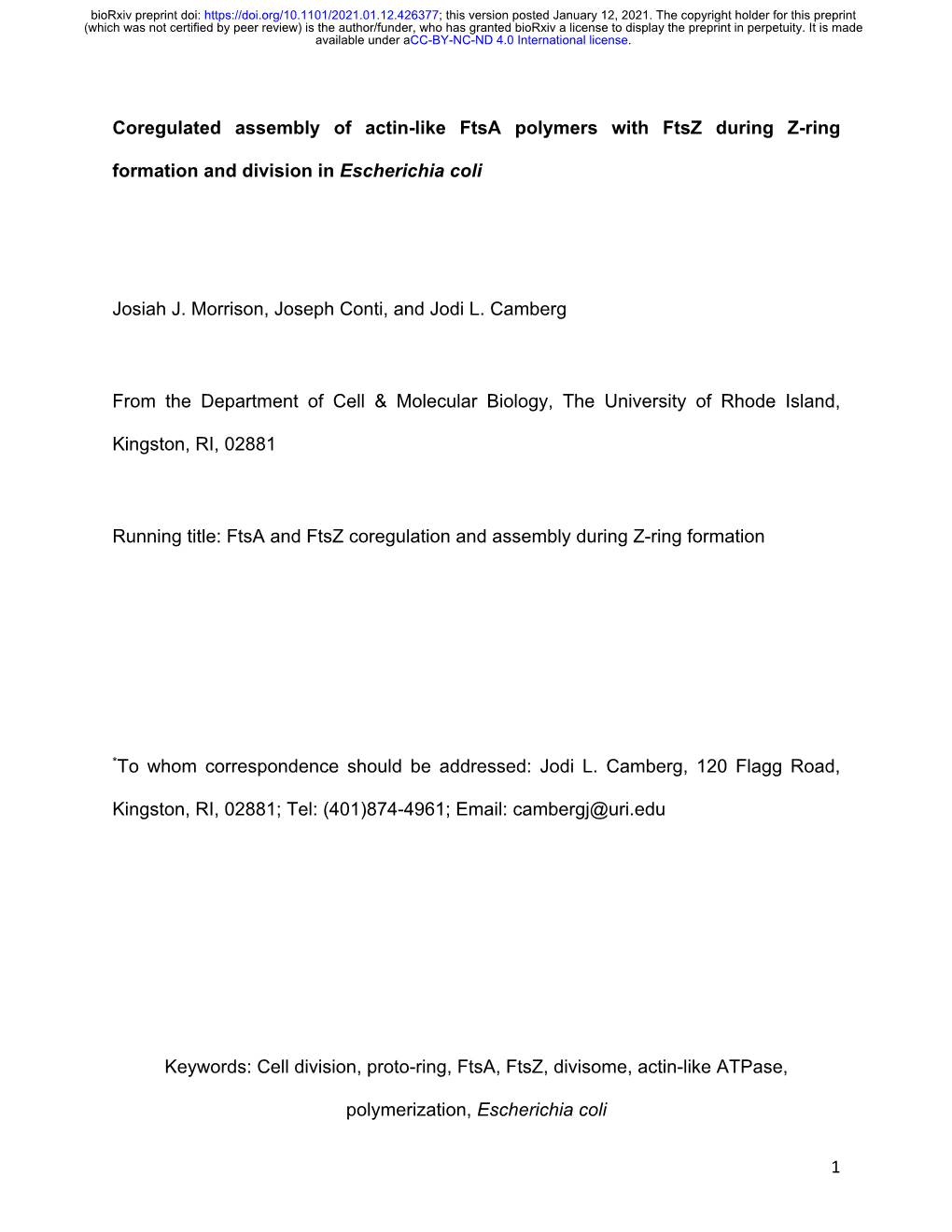
Load more
Recommended publications
-
Cell Division in Corynebacterineae
View metadata, citation and similar papers at core.ac.uk brought to you by CORE provided by Frontiers - Publisher Connector REVIEW ARTICLE published: 10 April 2014 doi: 10.3389/fmicb.2014.00132 Cell division in Corynebacterineae Catriona Donovan* and Marc Bramkamp* Department of Biology I, Ludwig-Maximilians-University, Munich, Planegg-Martinsried, Germany Edited by: Bacterial cells must coordinate a number of events during the cell cycle. Spatio-temporal Wendy Schluchter, University of regulation of bacterial cytokinesis is indispensable for the production of viable, genetically New Orleans, USA identical offspring. In many rod-shaped bacteria, precise midcell assembly of the division Reviewed by: machinery relies on inhibitory systems such as Min and Noc. In rod-shaped Actinobacteria, Julia Frunzke, Jülich Research Centre, Germany for example Corynebacterium glutamicum and Mycobacterium tuberculosis, the divisome Andreas Burkovski, Friedric assembles in the proximity of the midcell region, however more spatial flexibility is University of Erlangen-Nuremberg observed compared to Escherichia coli and Bacillus subtilis. Actinobacteria represent a (FAU), Germany group of bacteria that spatially regulate cytokinesis in the absence of recognizable Min and *Correspondence: Noc homologs. The key cell division steps in E. coli and B. subtilis have been subject to Catriona Donovan and Marc Bramkamp, Department of Biology I, intensive study and are well-understood. In comparison, only a minimal set of positive and Ludwig-Maximilians-University, negative regulators of cytokinesis are known in Actinobacteria. Nonetheless, the timing Munich, Großhaderner Str. 2-4, of cytokinesis and the placement of the division septum is coordinated with growth as 82152 Planegg-Martinsried, well as initiation of chromosome replication and segregation. -
Crystal Structure of Ftsa from Staphylococcus Aureus
FEBS Letters 588 (2014) 1879–1885 journal homepage: www.FEBSLetters.org Crystal structure of FtsA from Staphylococcus aureus Junso Fujita a, Yoko Maeda a, Chioko Nagao c, Yuko Tsuchiya c, Yuma Miyazaki a, Mika Hirose b, ⇑ Eiichi Mizohata a, Yoshimi Matsumoto d, Tsuyoshi Inoue a, Kenji Mizuguchi c, Hiroyoshi Matsumura a, a Department of Applied Chemistry, Graduate School of Engineering, Osaka University, 2-1 Yamadaoka, Suita, Osaka 565-0871, Japan b Department of Chemistry, Graduate School of Science, Osaka University, 1-1 Machikaneyama-cho, Toyonaka, Osaka 560-0043, Japan c National Institute of Biomedical Innovation, 7-6-8, Saito-Asagi, Ibaraki, Osaka 567-0085, Japan d Laboratory of Microbiology and Infectious Diseases, Institute of Scientific and Industrial Research, Osaka University, 8-1 Mihogaoka, Ibaraki, Osaka 567-0047, Japan article info abstract Article history: The bacterial cell-division protein FtsA anchors FtsZ to the cytoplasmic membrane. But how FtsA Received 5 February 2014 and FtsZ interact during membrane division remains obscure. We have solved 2.2 Å resolution crys- Revised 2 April 2014 tal structure for FtsA from Staphylococcus aureus. In the crystals, SaFtsA molecules within the dimer Accepted 2 April 2014 units are twisted, in contrast to the straight filament of FtsA from Thermotoga maritima, and the Available online 18 April 2014 half of S12–S13 hairpin regions are disordered. We confirmed that SaFtsZ and SaFtsA associate Edited by Kaspar Locher in vitro, and found that SaFtsZ GTPase activity is enhanced by interaction with SaFtsA. Structured summary of protein interactions: Keywords: Bacterial divisome SaFtsA and SaFtsZ bind by comigration in non denaturing gel electrophoresis (View interaction) Staphylococcus aureus SaFtsZ and SaFtsA bind by molecular sieving (View interaction) FtsA SaFtsA and SaFtsA bind by x-ray crystallography (View interaction) FtsZ Ó 2014 Federation of European Biochemical Societies. -
Structural and Genetic Analyses Reveal the Protein Sepf As a New
Structural and genetic analyses reveal the protein PNAS PLUS SepF as a new membrane anchor for the Z ring Ramona Dumana,1, Shu Ishikawab,1, Ilkay Celikc,1, Henrik Strahlc, Naotake Ogasawarab, Paulina Troca, Jan Löwea,2, and Leendert W. Hamoenc,d,2 aMedical Research Council Laboratory of Molecular Biology, Cambridge CB2 0QH, United Kingdom; bGraduate School of Information Science Functional Genomics, Nara Institute of Science and Technology, Ikoma, Nara 630-0101, Japan; cCentre for Bacterial Cell Biology, Institute for Cell and Molecular Biosciences, Newcastle University, Newcastle NE2 4AX, United Kingdom; and dSwammerdam Institute for Life Sciences, University of Amsterdam, 1098 XH, Amsterdam, The Netherlands Edited by Richard Losick, Harvard University, Cambridge, MA, and approved October 11, 2013 (received for review July 26, 2013) A key step in bacterial cell division is the polymerization of the N-terminal transmembrane domain (13). The necessity for ZipA tubulin homolog FtsZ at midcell. FtsZ polymers are anchored to the can be bypassed by a gain-of-function mutation in FtsA (14). cell membrane by FtsA and are required for the assembly of all Gram-positive bacteria contain EzrA, which shows a similar to- other cell division proteins. In Gram-positive and cyanobacteria, pology to that of ZipA, with an N-terminal transmembrane helix FtsZ filaments are aligned by the protein SepF, which in vitro pol- and a large C-terminal domain that binds to the FtsZ C terminus ymerizes into large rings that bundle FtsZ filaments. Here we de- (15). It therefore seemed likely that EzrA functions as an al- scribe the crystal structure of the only globular domain of SepF, ternative membrane anchor for the Z ring in B. -
Bacterial Actin Mreb Forms Antiparallel Double Filaments Fusinita Van Den Ent1*†, Thierry Izoré1†, Tanmay AM Bharat1, Christopher M Johnson2, Jan Löwe1
RESEARCH ARTICLE elifesciences.org Bacterial actin MreB forms antiparallel double filaments Fusinita van den Ent1*†, Thierry Izoré1†, Tanmay AM Bharat1, Christopher M Johnson2, Jan Löwe1 1Structural Studies Division, Medical Research Council - Laboratory of Molecular Biology, Cambridge, United Kingdom; 2Protein and Nucleic Acid Chemistry Division, Medical Research Council - Laboratory of Molecular Biology, Cambridge, United Kingdom Abstract Filaments of all actin-like proteins known to date are assembled from pairs of protofilaments that are arranged in a parallel fashion, generating polarity. In this study, we show that the prokaryotic actin homologue MreB forms pairs of protofilaments that adopt an antiparallel arrangement in vitro and in vivo. We provide an atomic view of antiparallel protofilaments of Caulobacter MreB as apparent from crystal structures. We show that a protofilament doublet is essential for MreB's function in cell shape maintenance and demonstrate by in vivo site-specific cross-linking the antiparallel orientation of MreB protofilaments in E. coli. 3D cryo-EM shows that pairs of protofilaments ofCaulobacter MreB tightly bind to membranes. Crystal structures of different nucleotide and polymerisation states of Caulobacter MreB reveal conserved conformational changes accompanying antiparallel filament formation. Finally, the antimicrobial agents A22/MP265 are shown to bind close to the bound nucleotide of MreB, presumably preventing nucleotide hydrolysis and destabilising double protofilaments. DOI: 10.7554/eLife.02634.001 *For correspondence: fent@mrc- lmb.cam.ac.uk Introduction †These authors contributed Cell shape is a characteristic and hereditary feature that is crucial for existence and its regulation is a equally to this work common challenge for organisms across all biological kingdoms. -
Cell Division in Escherichia Coli
Cell division in Escherichia coli Karl Skoog Cover: Homemade bread. Dividing. Baked by the author. ©Karl Skoog, Stockholm 2011 ISBN 978-91-7447-339-1, pp. 1-62 Printed in Sweden by US-AB, Stockholm 2011 Distributor: Department of Biochemistry and Biophysics, Stockholm University ii List of publications I Estimating Z-ring radius and contraction in dividing Escherichia coli. Strömqvist J, Skoog K, Daley DO, Widengren J, von Heijne G. Mol Microbiol. 2010 76(1):151-8 II Sequential closure of the cytoplasm then periplasm during cell division in Escherichia coli. Skoog K*, Söderström B*, Widengren J, von Heijne G, Daley DO. 2011 (Pending revision in J Bacteriol) III Penicillin-binding protein 5 can form a homo-oligomeric complex in the inner membrane of Escherichia coli. Skoog K, Stenberg Bruzell F, Ducroux A, Hellberg M, Johansson H, Lehtiö J, Högbom M, Daley DO. Protein Sci. 2011 20(9):1520-9. IV The Escherichia coli cell division protein ZipA forms homo-dimers prior to association with FtsZ. Skoog K, Daley DO. 2011. (submitted to Biochemistry) * These authors contributed equally to the work iii Abstract The Gram-negative bacterium Escherichia coli is a model system to describe the biochemistry and cell biology of cell division in bacteria. This process can be divided into three major steps. The first step involves the replication of the DNA, followed by an elongation step in which the cells become twice as long. In the last step the elongated cell constricts in the middle and the two daughter cells are separated. The cell division process in E. -
Cytoskeletal Proteins of Actinobacteria
Hindawi Publishing Corporation International Journal of Cell Biology Volume 2012, Article ID 905832, 10 pages doi:10.1155/2012/905832 Review Article Cytoskeletal Proteins of Actinobacteria Michal Letek, Marıa´ Fiuza, Almudena F. Villadangos, Luıs´ M. Mateos, and Jose´ A. Gil Instituto de Biolog´ıa Molecular, Genomica´ y Proteomica´ (INBIOMIC), Departamento de Biolog´ıa Molecular, Area´ de Microbiolog´ıa, Facultad de Biolog´ıa, Universidad de Leon,´ 24071 Leon,´ Spain Correspondence should be addressed to Jose´ A. Gil, [email protected] Received 28 July 2011; Revised 6 October 2011; Accepted 23 October 2011 Academic Editor: Timothy J. Yen Copyright © 2012 Michal Letek et al. This is an open access article distributed under the Creative Commons Attribution License, which permits unrestricted use, distribution, and reproduction in any medium, provided the original work is properly cited. Although bacteria are considered the simplest life forms, we are now slowly unraveling their cellular complexity. Surprisingly, not only do bacterial cells have a cytoskeleton but also the building blocks are not very different from the cytoskeleton that our own cells use to grow and divide. Nonetheless, despite important advances in our understanding of the basic physiology of certain bacterial models, little is known about Actinobacteria, an ancient group of Eubacteria. Here we review current knowledge on the cytoskeletal elements required for bacterial cell growth and cell division, focusing on actinobacterial genera such as Mycobacterium, Corynebacterium,andStreptomyces. These include some of the deadliest pathogens on earth but also some of the most prolific producers of antibiotics and antitumorals. 1. Introduction or murein), it was also recognized that other factors must drive the determination of bacterial cell shape [5]. -
Structural Determinants and Their Role in Cyanobacterial Morphogenesis
life Review Structural Determinants and Their Role in Cyanobacterial Morphogenesis Benjamin L. Springstein 1,* , Dennis J. Nürnberg 2 , Gregor L. Weiss 3 , Martin Pilhofer 3 and Karina Stucken 4 1 Department of Microbiology, Blavatnik Institute, Harvard Medical School, Boston, MA 02115, USA 2 Department of Physics, Biophysics and Biochemistry of Photosynthetic Organisms, Freie Universität Berlin, 14195 Berlin, Germany; [email protected] 3 Department of Biology, Institute of Molecular Biology & Biophysics, ETH Zürich, 8092 Zürich, Switzerland; [email protected] (G.L.W.); [email protected] (M.P.) 4 Department of Food Engineering, Universidad de La Serena, La Serena 1720010, Chile; [email protected] * Correspondence: [email protected] Received: 2 November 2020; Accepted: 9 December 2020; Published: 17 December 2020 Abstract: Cells have to erect and sustain an organized and dynamically adaptable structure for an efficient mode of operation that allows drastic morphological changes during cell growth and cell division. These manifold tasks are complied by the so-called cytoskeleton and its associated proteins. In bacteria, FtsZ and MreB, the bacterial homologs to tubulin and actin, respectively, as well as coiled-coil-rich proteins of intermediate filament (IF)-like function to fulfil these tasks. Despite generally being characterized as Gram-negative, cyanobacteria have a remarkably thick peptidoglycan layer and possess Gram-positive-specific cell division proteins such as SepF and DivIVA-like proteins, besides Gram-negative and cyanobacterial-specific cell division proteins like MinE, SepI, ZipN (Ftn2) and ZipS (Ftn6). The diversity of cellular morphologies and cell growth strategies in cyanobacteria could therefore be the result of additional unidentified structural determinants such as cytoskeletal proteins. -
The Role of Cytoskeletal Elements in Shaping Bacterial Cells Hongbaek Cho*
J. Microbiol. Biotechnol. (2015), 25(3), 307–316 http://dx.doi.org/10.4014/jmb.1409.09047 Research Article Review jmb The Role of Cytoskeletal Elements in Shaping Bacterial Cells Hongbaek Cho* Department of Microbiology and Immunobiology, Harvard Medical School, Boston, MA 02115, USA Received: September 16, 2014 Revised: September 26, 2014 Beginning from the recognition of FtsZ as a bacterial tubulin homolog in the early 1990s, many Accepted: September 26, 2014 bacterial cytoskeletal elements have been identified, including homologs to the major eukaryotic cytoskeletal elements (tubulin, actin, and intermediate filament) and the elements unique in prokaryotes (ParA/MinD family and bactofilins). The discovery and functional First published online characterization of the bacterial cytoskeleton have revolutionized our understanding of September 29, 2014 bacterial cells, revealing their elaborate and dynamic subcellular organization. As in *Corresponding author eukaryotic systems, the bacterial cytoskeleton participates in cell division, cell morphogenesis, Phone: +1-617-432-6970; DNA segregation, and other important cellular processes. However, in accordance with the Fax: +1-617-432-6970; vast difference between bacterial and eukaryotic cells, many bacterial cytoskeletal proteins E-mail: hongbaek_cho@hms. harvard.edu play distinct roles from their eukaryotic counterparts; for example, control of cell wall synthesis for cell division and morphogenesis. This review is aimed at providing an overview of the bacterial cytoskeleton, and discussing the roles and assembly dynamics of bacterial cytoskeletal proteins in more detail in relation to their most widely conserved functions, DNA pISSN 1017-7825, eISSN 1738-8872 segregation and coordination of cell wall synthesis. Copyright© 2015 by The Korean Society for Microbiology Keywords: Bacterial cytoskeleton, DNA segregation, FtsZ, MreB, ParA, peptidoglycan and Biotechnology Introduction cytokinesis, and cell morphogenesis. -
The Assembly and Interactions of Mreb in the Maintenance of Cell Shape in Caulobacter Crescentus
THE ASSEMBLY AND INTERACTIONS OF MREB IN THE MAINTENANCE OF CELL SHAPE IN CAULOBACTER CRESCENTUS A DISSERTATION SUBMITTED TO THE DEPARTMENT OF BIOCHEMISTRY AND THE COMMITTEE ON GRADUATE STUDIES OF STANFORD UNIVERSITY IN PARTIAL FULFILLMENT OF THE REQUIREMENTS FOR THE DEGREE OF DOCTOR OF PHILOSOPHY Natalie Anne Dye May, 2010 © 2010 by Natalie Anne Dye. All Rights Reserved. Re-distributed by Stanford University under license with the author. This work is licensed under a Creative Commons Attribution- Noncommercial 3.0 United States License. http://creativecommons.org/licenses/by-nc/3.0/us/ This dissertation is online at: http://purl.stanford.edu/bg008yn0701 ii I certify that I have read this dissertation and that, in my opinion, it is fully adequate in scope and quality as a dissertation for the degree of Doctor of Philosophy. Julie Theriot, Primary Adviser I certify that I have read this dissertation and that, in my opinion, it is fully adequate in scope and quality as a dissertation for the degree of Doctor of Philosophy. Lucille Shapiro, Co-Adviser I certify that I have read this dissertation and that, in my opinion, it is fully adequate in scope and quality as a dissertation for the degree of Doctor of Philosophy. James Spudich I certify that I have read this dissertation and that, in my opinion, it is fully adequate in scope and quality as a dissertation for the degree of Doctor of Philosophy. Aaron Straight Approved for the Stanford University Committee on Graduate Studies. Patricia J. Gumport, Vice Provost Graduate Education This signature page was generated electronically upon submission of this dissertation in electronic format. -
Do the Divisome and Elongasome Share a Common Evolutionary Past?, Curr Opin Microbiol (2013), J.Mib.2013.09.003
COMICR-1155; NO. OF PAGES 7 Available online at www.sciencedirect.com ScienceDirect Do the divisome and elongasome share a common evolutionary past? Piotr Szwedziak and Jan Lo¨ we The divisome and elongasome are bacterial protein complexes cytoplasm Lipid II, which carries the disaccharide-pep- responsible for peptidoglycan (PG) synthesis during cell tide building block for the polymerisation reaction. division and elongation, respectively. We review several lines of Lipid II is then flipped across the inner membrane into evidence, arguing for a shared evolutionary past of the the periplasm. PG polymerisation is facilitated by peni- divisome and elongasome. Both integrate closely related cillin-binding proteins (PBPs), which are DD-transpep- penicillin-binding proteins (PBPs) for PG synthesis, use tidases, DD-endopeptidases or DD-carboxypeptidases, proteins of the RodA/FtsW (SEDS, shape, elongation, division and some display transglycosylase activity as well. and sporulation) family for Lipid II export and interact with Hydrolases are needed for breaking bonds in order to MraY/Mur proteins for Lipid II synthesis. It was recently shown provide plasticity. Furthermore, dynamics of the inner that the actin-like protein FtsA of the divisome polymerises on membrane and PG layer have to be coordinated with the membranes, adding another parallel, since membrane- outer membrane in Gram-negative organisms. associated filaments of the bacterial actin MreB guide the elongasome. Given these similarities, it seems plausible to Here we discuss that the divisome and elongasome share conclude that the elongasome is a modified version of the features and subunits, arguing that they might have divisome, without the membrane-constricting FtsZ-ring and its descended from a common evolutionary ancestor. -
Bacterial Cytokinesis: from Z Ring to Divisome
REVIEW ARTICLE Cytoskeleton, October 2012 69:778–790 (doi: 10.1002/cm.21054) VC 2012 Wiley Periodicals, Inc. Bacterial Cytokinesis: From Z Ring to Divisome Joe Lutkenhaus, Sebastien Pichoff, and Shishen Du Department of Microbiology, Molecular Genetics and Immunology, University of Kansas Medical Center, Kansas City, Kansas Received 21 June 2012; Revised 18 July 2012; Accepted 20 July 2012 Monitoring Editor: Joseph Sanger Ancestral homologues of the major eukaryotic cytos- Cytokinesis in bacteria can be split into at least three keletal families, tubulin and actin, play critical roles in steps [de Boer, 2010]. First, is the assembly of the Z ring cytokinesis of bacterial cells. FtsZ is the ancestral on the cytoplasmic membrane with the aid of membrane homologue of tubulin and assembles into the Z ring tethering proteins [Pichoff and Lutkenhaus, 2002]. This that determines the division plane. FtsA, a member of step is under spatial and temporal control to ensure that the actin family, is involved in coordinating cell wall the Z ring is assembled between segregated chromosomes synthesis during cytokinesis. FtsA assists in the forma- [Lutkenhaus, 2007]. In the second step, which usually tion of the Z ring and also has a critical role in occurs after a considerable lag, the remaining cell division recruiting downstream division proteins to the Z ring proteins are added to the Z ring to form the complete to generate the divisome that divides the cell. Spatial divisome [Aarsman et al., 2005; Gamba et al., 2009; regulation of cytokinesis occurs at the stage of Z ring Goley et al., 2011]. Formation of this complex machine assembly and regulation of cell size occurs at this stage involves the addition of many essential proteins and an or during Z ring maturation. -
NIH Public Access Author Manuscript Nat Cell Biol
NIH Public Access Author Manuscript Nat Cell Biol. Author manuscript; available in PMC 2014 May 13. NIH-PA Author ManuscriptPublished NIH-PA Author Manuscript in final edited NIH-PA Author Manuscript form as: Nat Cell Biol. 2014 January ; 16(1): 38–46. doi:10.1038/ncb2885. The bacterial cell division proteins FtsA and FtsZ self-organize into dynamic cytoskeletal patterns Martin Loose* and Timothy J. Mitchison Department of Systems Biology, Harvard Medical School, 200 Longwood Avenue, Boston, MA 02115, USA Abstract Bacterial cytokinesis is commonly initiated by the Z-ring, a cytoskeletal structure assembling at the site of division. Its primary component is FtsZ, a tubulin superfamily GTPase, which is recruited to the membrane by the actin-related protein FtsA. Both proteins are required for the formation of the Z-ring, but if and how they influence each other’s assembly dynamics is not known. Here, we reconstituted FtsA-dependent recruitment of FtsZ polymers to supported membranes, where both proteins self-organize into complex patterns, such as fast-moving filament bundles and chirally rotating rings. Using fluorescence microscopy and biochemical perturbations, we found that these large-scale rearrangements of FtsZ emerge from its polymerization dynamics and a dual, antagonistic role of FtsA: recruitment of FtsZ filaments to the membrane and a negative regulation on FtsZ organization. Our findings provide a model for the initial steps of bacterial cell division and illustrate how dynamic polymers can self-organize into large-scale structures. As in eukaryotic cells, proteins related to actin and tubulin provide the key structural components coordinating cellular functions in bacteria1,2.