Spatial Coincidence and Similar Geochemistry of Late Triassic And
Total Page:16
File Type:pdf, Size:1020Kb
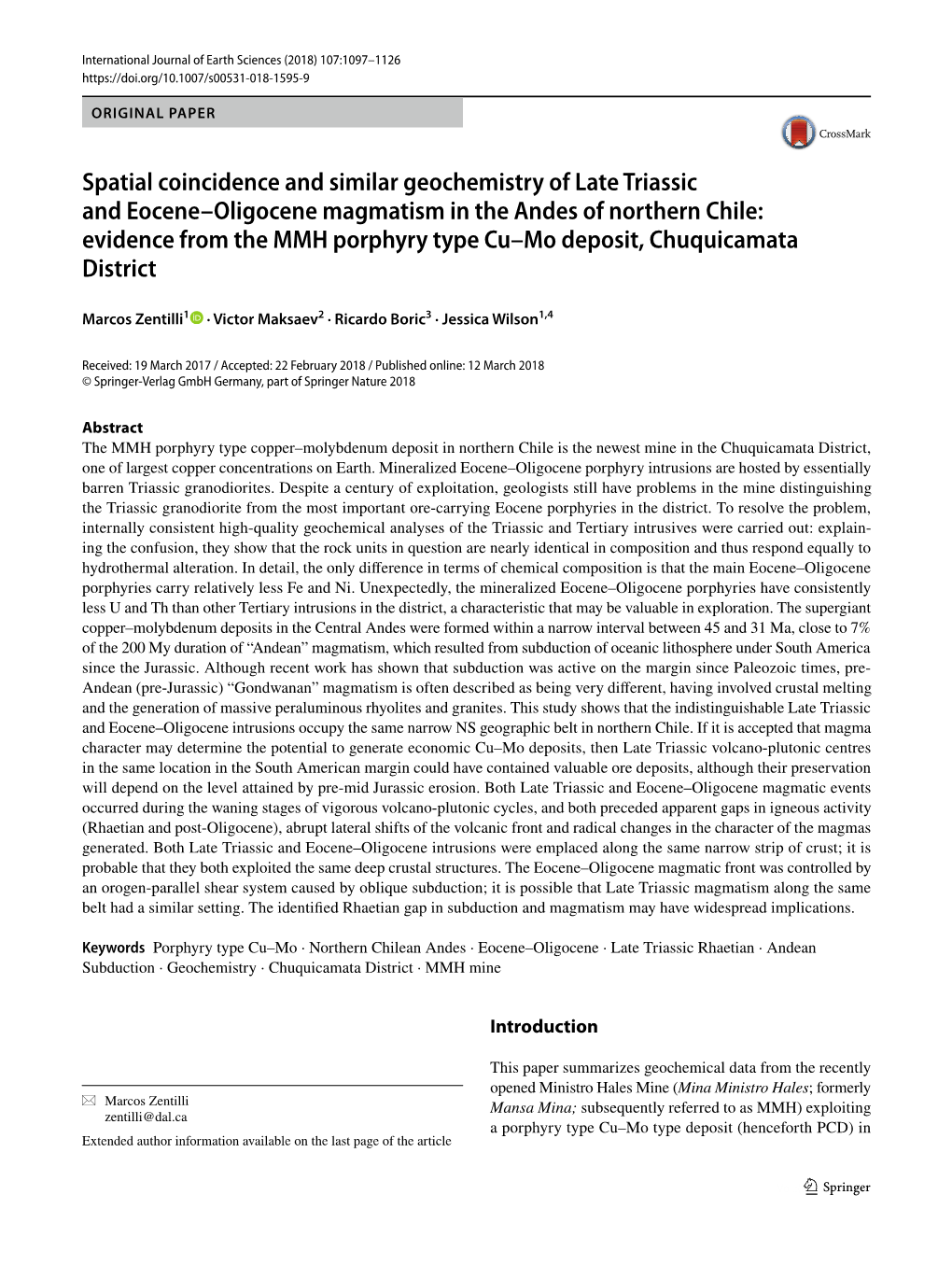
Load more
Recommended publications
-
Counterclockwise Rotation of Late Eocene – Oligocene Forearc Deposits in Southern Peru and Its Significance for Oroclinal Bend
Counterclockwise rotation of Late Eocene – Oligocene forearc deposits in southern Peru and its significance for oroclinal bending in the Central Andes Pierrick Roperch, Thierry Sempere, Orlando Macedo, César Arriagada, Michel Fornari, Claudio Tapia, Marcelo Garcia, Carlo Laj To cite this version: Pierrick Roperch, Thierry Sempere, Orlando Macedo, César Arriagada, Michel Fornari, et al.. Coun- terclockwise rotation of Late Eocene – Oligocene forearc deposits in southern Peru and its significance for oroclinal bending in the Central Andes. Tectonics, American Geophysical Union (AGU), 2006, 25 (3), pp.TC3010. 10.1029/2005TC001882. insu-00261209 HAL Id: insu-00261209 https://hal-insu.archives-ouvertes.fr/insu-00261209 Submitted on 29 Jun 2016 HAL is a multi-disciplinary open access L’archive ouverte pluridisciplinaire HAL, est archive for the deposit and dissemination of sci- destinée au dépôt et à la diffusion de documents entific research documents, whether they are pub- scientifiques de niveau recherche, publiés ou non, lished or not. The documents may come from émanant des établissements d’enseignement et de teaching and research institutions in France or recherche français ou étrangers, des laboratoires abroad, or from public or private research centers. publics ou privés. TECTONICS, VOL. 25, TC3010, doi:10.1029/2005TC001882, 2006 Counterclockwise rotation of late Eocene–Oligocene fore-arc deposits in southern Peru and its significance for oroclinal bending in the central Andes Pierrick Roperch,1,2 Thierry Sempere,3 Orlando Macedo,4 Ce´sar Arriagada,2 Michel Fornari,5 Claudio Tapia,2 Marcelo Garcı´a,6 and Carlo Laj7 Received 6 July 2005; revised 20 February 2006; accepted 14 March 2006; published 1 June 2006. -
Late Mesozoic to Paleogene Stratigraphy of the Salar De Atacama Basin, Antofagasta, Northern Chile: Implications for the Tectonic Evolution of the Central Andes
Late Mesozoic to Paleogene stratigraphy of the Salar de Atacama Basin, Antofagasta, Northern Chile: Implications for the tectonic evolution of the Central Andes Constantino Mpodozisa,T,Ce´sar Arriagadab, Matilde Bassoc, Pierrick Roperchd, Peter Cobbolde, Martin Reichf aServicio Nacional de Geologı´a y Minerı´a, now at Sipetrol. SA, Santiago, Chile bDepartamento de Geologı´a, Universidad de Chile, Santiago, Chile cServicio Nacional de Geologı´a y Minerı´a, Santiago, Chile dIRD/Dep. de Geologı´a, Universidad de Chile, Santiago, Chile eGe´osciences-Rennes (UMR6118 du CNRS), France fDepartment of Geological Sciences University of Michigan, United States Abstract The Salar de Atacama basin, the largest bpre-AndeanQ basin in Northern Chile, was formed in the early Late Cretaceous as a consequence of the tectonic closure and inversion of the Jurassic–Early Cretaceous Tarapaca´ back arc basin. Inversion led to uplift of the Cordillera de Domeyko (CD), a thick-skinned basement range bounded by a system of reverse faults and blind thrusts with alternating vergence along strike. The almost 6000-m-thick, upper Cretaceous to lower Paleocene sequences (Purilactis Group) infilling the Salar de Atacama basin reflects rapid local subsidence to the east of the CD. Its oldest outcropping unit (Tonel Formation) comprises more than 1000 m of continental red sandstones and evaporites, which began to accumulate as syntectonic growth strata during the initial stages of CD uplift. Tonel strata are capped by almost 3000 m of sandstones and conglomerates of western provenance, representing the sedimentary response to renewed pulses of tectonic shortening, which were deposited in alluvial fan, fluvial and eolian settings together with minor lacustrine mudstone (Purilactis Formation). -
Crustal Faults in the Chilean Andes: Geological Constraints and Seismic Potential
Andean Geology 46 (1): 32-65. January, 2019 Andean Geology doi: 10.5027/andgeoV46n1-3067 www.andeangeology.cl Crustal faults in the Chilean Andes: geological constraints and seismic potential *Isabel Santibáñez1, José Cembrano2, Tiaren García-Pérez1, Carlos Costa3, Gonzalo Yáñez2, Carlos Marquardt4, Gloria Arancibia2, Gabriel González5 1 Programa de Doctorado en Ciencias de la Ingeniería, Pontificia Universidad Católica de Chile, Avda. Vicuña Mackenna 4860, Macul, Santiago, Chile. [email protected]; [email protected] 2 Departamento de Ingeniería Estructural y Geotécnica, Pontificia Universidad Católica de Chile, Avda. Vicuña Mackenna 4860, Macul, Santiago, Chile. [email protected]; [email protected]; [email protected] 3 Departamento de Geología, Universidad de San Luis, Ejercito de Los Andes 950, D5700HHW San Luis, Argentina. [email protected] 4 Departamento de Ingeniería Estructural y Geotécnica y Departamento de Ingeniería de Minería, Pontificia Universidad Católica de Chile. Avda. Vicuña Mackenna 4860, Macul, Santiago, Chile. [email protected] 5 Departamento de Ciencias Geológicas, Universidad Católica del Norte, Angamos 0610, Antofagasta, Chile. [email protected] * Corresponding author: [email protected] ABSTRACT. The Chilean Andes, as a characteristic tectonic and geomorphological region, is a perfect location to unravel the geologic nature of seismic hazards. The Chilean segment of the Nazca-South American subduction zone has experienced mega-earthquakes with Moment Magnitudes (Mw) >8.5 (e.g., Mw 9.5 Valdivia, 1960; Mw 8.8 Maule, 2010) and many large earthquakes with Mw >7.5, both with recurrence times of tens to hundreds of years. By contrast, crustal faults within the overriding South American plate commonly have longer recurrence times (thousands of years) and are known to produce earthquakes with maximum Mw of 7.0 to 7.5. -
Plate Tectonic Setting of the Andean Cordillera
183 by Victor A. Ramos Plate tectonic setting of the Andean Cordillera Laboratorio de Tectónica Andina, Universidad de Buenos Aires, Argentina. E-mail: [email protected] The Andes are a natural laboratory for the study of the acterize the different segments are widely variable. The present interaction between subduction of the oceanic plate and overview will focus on the major geological differences among these segments, based on today’s plate-tectonic knowledge of this moun- active geological processes. Inter- and intraplate seis- tain chain. micity, volcanic activity, thick- and thin-skinned fold and thrust belts, and foreland basin subsidence, in con- junction with space geodetic observations, contribute to Major geological provinces characterize the present plate tectonic setting of discrete The Andes north of the Golfo de Guayaquil are unique, as estab- segments of the Andes. The inherited geological history, lished by Gansser (1973). The Northern Andes record an important as well as the present tectonic setting, is responsible for accretion of oceanic crust during Jurassic, late Cretaceous, and Pale- the unique geology of the Northern, Central, and South- ogene times. As a result, the Western Cordillera of Colombia and Ecuador is mainly constituted of an oceanic basement that during ern Andes. The Northern Andes are the result of Meso- accretion was related to ophiolite obduction, important penetrative zoic and Cenozoic collisions of oceanic terranes, prior deformation and metamorphism, in cases up to blue schist facies. to the present Andean-type setting. The Central Andes Further north, the emplacement of the Caribbean nappes was related to the collision of an island arc system, the Bonaire block, during have a long history of subduction and volcanic arc Paleogene times (Bosch and Rodríguez, 1992, Kellogg and Bonini, activity, while the Southern Andes record the closing of 1982). -
North Chilean Andes: Orogenic Implications
6th International Symposium on Andean Geodynamics (ISAG 2005, Barcelona), Extended Abstracts: 548-551 Thermochronological data and denudation history along a transect between Chaüaral and Pedernales (- 26°5), North Chilean Andes: Orogenic implications Thierry Nalpas (1,5), Gérard Hérail (1), Constantino Mpodozis (2), Rodrigo Riquelme (3), Jorge Clavero (4), & Marie-Pierre Dabard (5) (1) IRD -lMTG , Roman Diaz 264, Casilla 53390, Correo Central, Santiago, Chile, and Departamento de Geologia , Facultad de Ciencias Fisicas y Matematicas de la Universidad de Chile. Santiago , Chile (2) SIPETROl, Av. Vitacura 2736, las Condes, Santiago de Chile , Chile (3) Universidad Catôlica del Norte, Avenida Angamos 0610, Antofagasta, Chile (4) SERNAGEOMIN, Av Santa Maria 0104, Providencia, Santiago, Chile (5) Géosciences Rennes, Université de Rennes 1, Campus de Beaulieu , 35042 Rennes cedex , France KEYWORDS: erosion, denudation , orogenesis, Andes, (hile Introduction The Chilean Andes along the southern Atacama Desert (26°-27°S) include into four main morphotectonic units : the Coastal Cordillera and the Central Valley, Precordillera (or Cordillera de Domeyko), Preandean depression (Pedernales-Maricunga basins) and Western Cordillera (Figure 1). Within the studied region, the second order Cordillera Claudio Gay separates the Western Cordillera from the Preandean depression. 25' P:Pedemales salar M:Marlcungasalar y mainvalley 72" 71' Figure 1: Localisation of the studied area (Salar de Pedernales P, y Maricunga M). From the coast to the Mar icunga-Pedernales -
Eocene Left Lateral Strike Slip Faulting and Clockwise Block Rotations in The
Second ISAG, Oxford (UK),21-231911993 225 EOCENELEFT LATERAL STRIKESLIP FAULTlNG AND CLOCKWISEBLOCK ROTATIONS IN THE CORDILLEIZA DE DOMEYKO, WEST OF SALAK DE ATACAMA,NORTHERN CHILE Constantin0 MPODOZIS(I), Nicolas MAlUNOVIC(l),Ingrid SMOJE(1) (1) Servicio Nacionalde Geologia y Mineria, Avda. Santa Maria0104, Santiago, Chile RESUMEN: La Cordillera de Domeyko,al oeste de Salar de Atacama, en el norte de Chile, constituye parte de un or6geno transcurrente, originado en el Eoceno, por movimientos de rumbo sinistrales a 10 largo del Sistema de Fallas de Domeyko. En el segmento comprendido cntrcel Salar dePunk? Negra, al sur y Sierra de Limdn Verde, al nortc, el movimiento hacia el norte de la Cordillera de Domeyko fue bloqueado por la presencia de una mole rigida (Sierra Lim6n Verde);y transferido hacia el este por rotaciones de bloques en sentido horario, endireccih a la la Cuenca cretQcicade Purilactis (prolo salar de Atacama), la InQsoccidental de las cuencas de riftcretkicas del noroeste de Argentina y norte de Chile. La inusual co~nbinacidnde rotaciones horarias acompaiiando a movimientos transcurrentes sinistrales se deberfa a que el sistema transcurrente sinistral estuvo limitadoal norte por una mole rigiday a al este, por una cara libre:la Cuenca de Purilactis. Key Words: Northern Chile, Domeyko Fault System, Eocene, Clockwise block rotations INTRODUCTION Cordillera de Domeyko and its northeni prolongation, Sierrade Moreno, extends for moreUlan 800 km (21-28"s) in Northern Chile (Figure 1) sepvating the Central Depression, to Ule West, from a series of closed basins ( Salar de Atacama, Punta Negra, Pedeniales etc) located along the footllills of the western Cordillera, thatis capped by the volcanic centersof the modem volcanic arc of tl~eCentral Andes. -
Sciencedirect.Com Sciencedirect
Available online at www.sciencedirect.com ScienceDirect Geochimica et Cosmochimica Acta 135 (2014) 29–48 www.elsevier.com/locate/gca Geochemical, isotopic, and mineralogical constraints on atmospheric deposition in the hyper-arid Atacama Desert, Chile Fan Wang a, Greg Michalski a,⇑, Ji-hye Seo b, Wensheng Ge c a Department of Earth, Atmospheric and Planetary Sciences, Purdue University, West Lafayette, IN 47907, USA b Department of Chemistry, Purdue University, West Lafayette, IN 47907, USA c School of Earth Sciences and Resources, China University of Geosciences, Beijing, China Received 12 September 2013; accepted in revised form 14 March 2014; available online 26 March 2014 Abstract Modern atmospheric deposition across the Atacama was collected by an array of dust traps that stretched from the Pacific coast to the Andean altiplano, and the material was analyzed for its geochemical, mass and isotopic composition. The coastal trap had the second-highest insoluble mineral particle and highest soluble salt deposition rates due to significant inputs from the Morro Mejillones Range and the Pacific Ocean, respectively. The Andean trap had the highest insoluble mineral particle deposition owing to transport of weathered material, but the lowest deposition rate of soluble salts due to its distance from the ocean and anthropogenic sources. The removal of oceanic material was effective by the coastal mountains, while the westward transport of the Andean material was determined to be minimal. The atmospheric deposition in the inland traps was mainly from the local entrainment of surface material, inland anthropogenic emissions, and transport of marine aerosols. The nitrate 15 17 À isotopes (d N and D O) suggested that NOx sources and NO3 chemistry shifted along the west–east transect, and were À greatly impacted by anthropogenic emissions with soil NO3 being a minor source of deposited nitrogen. -
Internal Structure and Petrography of the Mineralized Faults in the Radomiro Tomic Deposit
O EOL GIC G A D D A E D C E I H C I L E O S F u n 2 d 6 la serena octubre 2015 ada en 19 Internal Structure and Petrography of the Mineralized Faults in the Radomiro Tomic Deposit Erik Jensen*, Gabriel González, Facultad de Ingeniería y Ciencias Geológicas, Universidad Católica del Norte, Avenida Angamos 0610, Antofagasta, Chile *Contact email: [email protected] Abstract. The interaction between faults and hydrothermal largest ore-bearing fault systems in the world (DFS). fluids is studied observing the internal characteristics of the Aimed to unravel the temporal and genetic relationship faults in RT deposit. Distribution and petrography of fault- between faulting and fluid-induced alteration, in order to related rocks, grouped in units (studied with optical understand their effects on the evolution of the crustal microscope and XRD) unravel their timing and genesis. rheology. Subsequently, nature of pore fluids and evolution of mechanical properties of faults are deduced. Both rock deformation and circulation of fluids in the deposit Case of study were conducted through the successive reutilization of the same structures (N30-60E/~90). Initially quartz veins and The case of study RT, is located within a fore-arc trench- afterwards fault planes. During the nucleation of faults, parallel fault system, called Domeyko Fault System (DFS). phyllic fluids permeated the deposit, adding pyrite The location of several porphyry deposits along it suggest (+chalcopyrite) to the fractures and transforming feldspar to high permeation of hydrothermal fluids trough the crust, at illite in the haloes. Subsequently, these faults were sheared 3-5 km depth (subsequently exhumed). -
Chapter 3 Geologic Overview of the Escondida Porphyry Copper District, Northern Chile
© 2012 Society of Economic Geologists, Inc. Special Publication 16, pp. 55–78 Chapter 3 Geologic Overview of the Escondida Porphyry Copper District, Northern Chile MIGUEL HERVÉ1,* RICHARD H. SILLITOE,2 CHILONG WONG,1 PATRICIO FERNÁNDEZ,1,** FRANCISCO CRIGNOLA,1,*** MARCO IPINZA,1 AND FELIPE URZÚA3 1 Minera Escondida Limitada, Avenida de la Minería 501, Antofagasta, Chile 2 27 West Hill Park, Highgate Village, London N6 6ND, England 3 BHP Billiton, 10 Marina Boulevard 50-01, Marina Bay Financial Centre Tower 2, Singapore 018983 Abstract The giant Escondida district in northern Chile, discovered in 1981, includes the major porphyry copper de- posits at Escondida-Escondida Este, Escondida Norte-Zaldívar, Pampa Escondida, and two small deposits (the Escondida cluster), besides the Chimborazo deposit. The district contains at least 144 million metric tons (Mt) of copper. The Escondida district is part of the middle Eocene to early Oligocene porphyry copper belt, which follows the trench-parallel Domeyko fault system, a product of the Incaic transpressional tectonic phase. At the district scale, the major N-striking Portezuelo-Panadero oblique-reverse fault juxtaposes latest Carboniferous to Early Permian igneous basement with an andesitic volcanic sequence of late Paleocene to early Eocene age, both of which host the porphyry copper mineralization. Immediately before and during porphyry copper for- mation, a thick siliciclastic sequence with andesitic volcanic products intercalated toward the top (San Carlos strata) filled a deep basin, generated by clockwise rigid-block rotation, within the confines of the Escondida cluster. The presence of these volcanic rocks suggests that an eruptive center was still active within the con- fines of the Escondida cluster when deposit formation began. -
Falla Oeste Fault System : Record of Its Regional Significance As Exposed in the Chuquicamata Open Pit, Northern Chile
Third ISAG, St Malo (France), 17-19/9/1996 FALLA OESTE FAULT SYSTEM : RECORD OF ITS REGIONAL SIGNIFICANCE AS EXPOSED IN THE CHUQUICAMATA OPEN PIT, NORTHERN CHILE Darryl D. Lindsay"', Marcos Zentilli") and Guillermo Ossandon'" (1) Department of Earth Sciences, Dalhousie University, Halifax, NS, B3H 3J5, Canada (2) Superintendencia de Geologia, CODELCO- Chuquicamata, Chuquicamata, Chile KEY WORDS : tectonics, extension, strike-slip faults, left-lateral, fault gouge, mineralization INTRODUCTION Late Eocene subduction related ma_matic arc tectonics produced orogen-normal shortening and dextral orogen-parallel strike-slip motions in the Precordillera of northern Chile (Scheuber and Reutter, 1992). This Incaic tectonic phase, within the Precordillera, formed the Domeyko Fault System along which numerous Eocene- Oligocene intrusive complexes bearing porphyry copper mineralization were emplaced, The Falla Oeste is often referred to as the essential branch of the Domeyko (Maksaev, 1990) or Precordilleran (Reuner et al., 1991) Fault System in northern Chile. The Falla Oeste is best defined as a fault system of regional scales ,with individual branches or sub-systems of faults that record approximately 40 m.y. of regional deformation. Within the Chuquicamata district the Falla Oeste fault system is comprised of the Mesabi, Estanques Blancos, Balmaceda, Nor-Oeste, Este, Americana, Calderones, West Fissure, Chucos, San Lorenzo, Zaragoza, C2 and several important un-named faults and fault systems. The regionally important Falla Oeste, which has tectonic significance, must be differentiated fIom the district scale fault that traverses the Chuquicamata open pit, herein, the West Fissure, as displayed on original district and deposit scale maps (see Lopez, 1939). Differences in the kinematics of the Domeyko fault system (right-lateral, unknown total displacement) with those of the West Fissure (left-lateral, estimated 35-40 km (Ambrus, 1979)) have been explained by a tectonic inversion of the fault system (Reuner et al., 1993). -
Elements 11: 91-98
Arc Magmatic Tempos: Gathering the Evidence Paterson and Ducea (2015) Elements 11: 91-98 Sources of data presented in figures (listed by figure number) Figure 1 Data Sources Bedrock ages Chapman AD, Saleeby JB, Wood DJ, Piasecki A, Farley KA, Kidder S, Ducea MN (2012) Late Cretaceous gravitational collapse of the southern Sierra Nevada batholith, California. Geosphere 8: 314–341 Detrital ages Unpublished data from SR Paterson Figure 2 Data Sources Bedrock ages Aguirre-Urreta MB, Pazos PJ, Lazo DG, Fanning CM, Litvak VD (2008) First U-Pb SHRIMP age of the Hauterivian 91-99 Alanizstage,-Álvarez Neuquén SA, van Basin, der Heyden Argentina. P, Samaniego Journal of SouthAFN, Ortega American- Earth Sciences 26: evidence for Middle Jurassic strike-slip faulting in southern Mexico related to the opening of the Gulf of Mexico: Geology 24: 443– Gutiérrez F (1996) Radiometric and kinematic Alasino PH and 7 coauthors (2012)446 Early Carboniferous sub- to mid-alkaline magmatism in the Eastern Sierras Pampeanas, NW Argentina: A record of crustal growth by the incorporation of mantle-derived material in an extensional setting: Gondwana Research 22: 992–1008 Provenance, volcanic record, and tectonic setting of the Paleozoic Ventania Fold Belt and the Claromecó Foreland Basin: Implications on sedimentation and volcanism along Alessandrettithe southwestern L and 6 coauthors Gondwana (2013) margin. Journal of South American Earth Sciences 47: 12–31 Alldrick DJ (2001) Geology and mineral deposits of the Ecstall belt, northwest British Columbia, in Geological Fieldwork 1999, Paper 2000-1, BC Ministry of Energy and Mines 2: 8279– Álvarez J and 8 coauthors (2011) Detrital zircons from late Paleozoic accretionary306 complexes in north-central Chile (28°–32°S): Possible fingerprints of the Chilenia. -
Pampa Metals Initiates Geophysical Program at Its Arrieros Copper Project in Chile
PM CSE www.pampametals.com Pampa Metals Initiates Geophysical Program at its Arrieros Copper Project in Chile ________________________________________________________________________ (CSE: PM) (FSE: FIRA) For Immediate Release Vancouver – December 23, 2020 – Pampa Metals Corp. (“Pampa Metals” or the “Company”) (CSE: PM) is pleased to announce that the company has initiated geophysical surveying at its Arrieros copper project in northern Chile. Pampa Metals has started to acquire Resistivity and 3D Vector Induced Polarization (“VIP”), together with Magneto-Telluric (“MT”) and ground magnetic measurements over the 14,000-hectare property, which is characterised by extensive post-mineral gravel cover and is located along the preeminent Eocene-Oligocene porphyry copper belt of northern Chile. Julian Bavin, CEO of Pampa Metals said: “We are very pleased that we have now initiated trading on the Canadian Securities Exchange (“CSE”) as announced a week ago (see News Release of December 14, 2020). We have immediately put our treasury money to work, have executed the geophysical contract for the work program at both Arrieros and Redondo-Veronica projects, and are now undertaking geophysical surveying at Arrieros. We are looking forward to a busy work program in 2021.” Geophysical data acquisition is being carried out by SouthernRock Geophysics (“SRG”), an experienced Chile-based geophysical contractor with decades of experience in the acquisition of geophysical data in the conditions imposed by the Atacama Desert of northern Chile. Fieldwork started 10 days ago with the excavation of pits for transmitter electrode contacts. SRG has determined that the ground electrical contacts tested are apt for injection of adequate electrical current into the caliche and gravel cover for optimum survey conditions, and VIP and MT data acquisition is now in progress.