Microbead-Based Biosensing in Microfluidic Devices
Total Page:16
File Type:pdf, Size:1020Kb
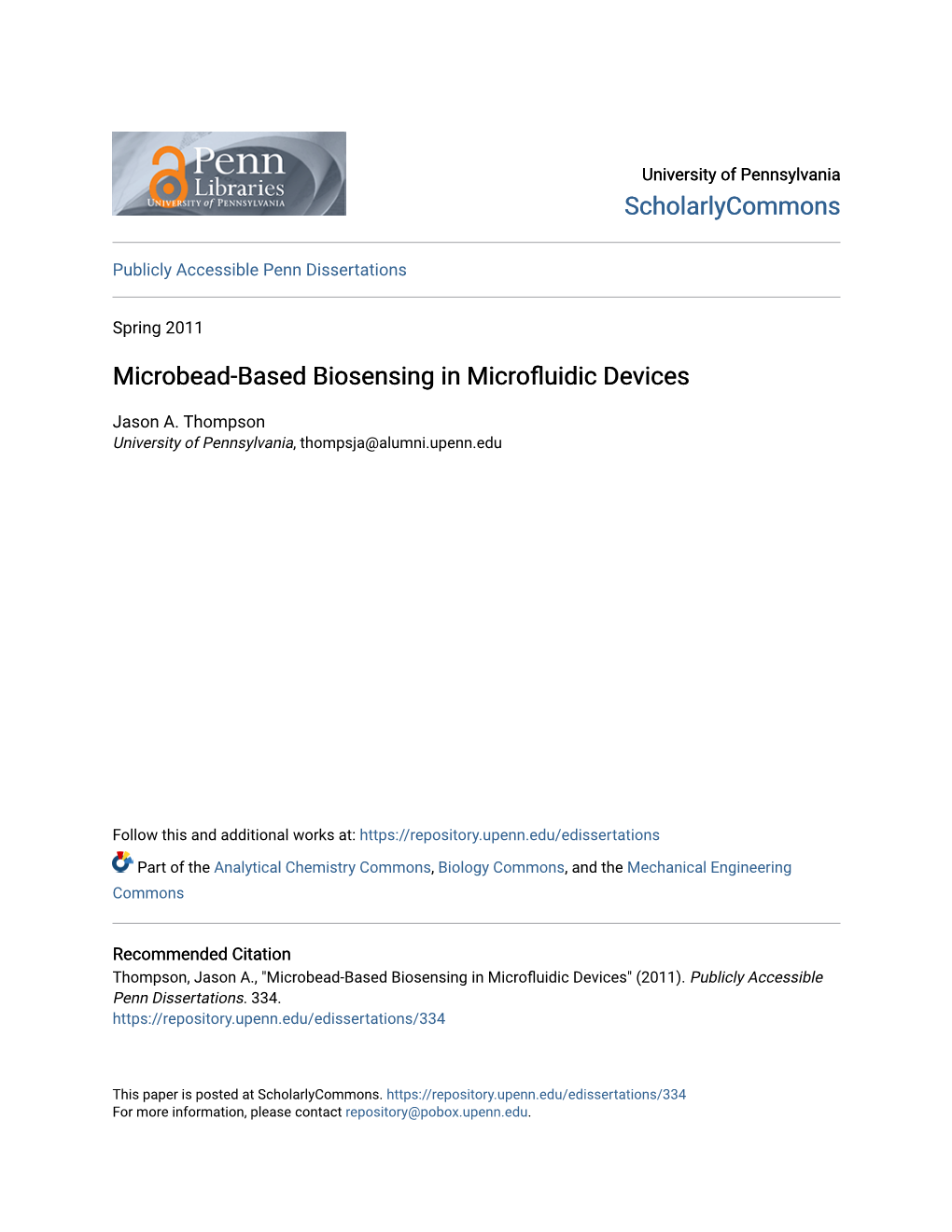
Load more
Recommended publications
-
An Assessment Report on Microplastics
An Assessment Report on Microplastics This document was prepared by B Stevens, North Carolina Coastal Federation Table of Contents What are Microplastics? 2 Where Do Microplastics Come From? 3 Primary Sources 3 Secondary Sources 5 What are the Consequences of Microplastics? 7 Marine Ecosystem Health 7 Water Quality 8 Human Health 8 What Policies/Practices are in Place to Regulate Microplastics? 10 Regional Level 10 Outer Banks, North Carolina 10 Other United States Regions 12 State Level 13 Country Level 14 United States 14 Other Countries 15 International Level 16 Conventions 16 Suggested World Ban 17 International Campaigns 18 What Solutions Already Exist? 22 Washing Machine Additives 22 Faucet Filters 23 Advanced Wastewater Treatment 24 Plastic Alternatives 26 What Should Be Done? 27 Policy Recommendations for North Carolina 27 Campaign Strategy for the North Carolina Coastal Federation 27 References 29 1 What are Microplastics? The category of ‘plastics’ is an umbrella term used to describe synthetic polymers made from either fossil fuels (petroleum) or biomass (cellulose) that come in a variety of compositions and with varying characteristics. These polymers are then mixed with different chemical compounds known as additives to achieve desired properties for the plastic’s intended use (OceanCare, 2015). Plastics as litter in the oceans was first reported in the early 1970s and thus has been accumulating for at least four decades, although when first reported the subject drew little attention and scientific studies focused on entanglements, ‘ghost fishing’, and ingestion (Andrady, 2011). Today, about 60-90% of all marine litter is plastic-based (McCarthy, 2017), with the total amount of plastic waste in the oceans expected to increase as plastic consumption also increases and there remains a lack of adequate reduce, reuse, recycle, and waste management tactics across the globe (GreenFacts, 2013). -
(WHO) Report on Microplastics in Drinking Water
Microplastics in drinking-water Microplastics in drinking-water ISBN 978-92-4-151619-8 © World Health Organization 2019 Some rights reserved. This work is available under the Creative Commons Attribution-NonCommercial-ShareAlike 3.0 IGO licence (CC BY-NC-SA 3.0 IGO; https://creativecommons.org/licenses/by-nc-sa/3.0/igo). Under the terms of this licence, you may copy, redistribute and adapt the work for non-commercial purposes, provided the work is appropriately cited, as indicated below. In any use of this work, there should be no suggestion that WHO endorses any specific organization, products or services. The use of the WHO logo is not permitted. If you adapt the work, then you must license your work under the same or equivalent Creative Commons licence. If you create a translation of this work, you should add the following disclaimer along with the suggested citation: “This translation was not created by the World Health Organization (WHO). WHO is not responsible for the content or accuracy of this translation. The original English edition shall be the binding and authentic edition”. Any mediation relating to disputes arising under the licence shall be conducted in accordance with the mediation rules of the World Intellectual Property Organization. Suggested citation. Microplastics in drinking-water. Geneva: World Health Organization; 2019. Licence: CC BY-NC-SA 3.0 IGO. Cataloguing-in-Publication (CIP) data. CIP data are available at http://apps.who.int/iris. Sales, rights and licensing. To purchase WHO publications, see http://apps.who.int/bookorders. To submit requests for commercial use and queries on rights and licensing, see http://www.who.int/about/licensing. -
4 S2 2 Box&Cummins-Policy Talk
Data Supported Solutions for Microplastics Pollution in San Francisco Bay Carolynn Box and Anna Cummins 5 Gyres Research supports the plastic pollution movement Research often backbone to action campaigns Local data can support local policy action Example: microbead ban in California This project was designed to develop scientifically supported solutions Policy Expert Committee • 22 experts / stakeholders • Diverse group of views Policy Recommendation Report • Based on project results 1 Support policies that reduce single-use plastics and packaging Local: San Francisco and others are looking to Berkeley’s disposable free ordinance Statewide: Comprehensive legislation that reduces single-use plastics 1 Support policies that reduce single-use plastics and packaging Project results • Surface water had polyethylene and polypropylene fragments, polystyrene foam • Computer modeling shows that buoyant particles are transported out of Bay Explore green stormwater 2 infrastructure as a tool to reduce microplastics Green stormwater infrastructure • Remove hard surfaces to reduce runoff to nearby water bodies Related study by SFEI • 90% removal of microplastics • Maintenance is ongoing Explore green stormwater 2 infrastructure as a tool to reduce microplastics Project results • 7 trillion microplastics enter through stormwater, 300 times more than wastewater • Half of the particles were rubbery black fragments, likely linked to tires More research on stormwater is needed 3 Identify and prioritize intervention points for fibers by filtration Evaluate -
MICROPLASTICS PROJECT Science-Supported Solutions and Policy Recommendations
SAN FRANCISCO BAY MICROPLASTICS PROJECT Science-Supported Solutions and Policy Recommendations Authors: Carolynn Box (5 Gyres) Anna Cummins (5 Gyres) September 2019 CONTENTS EXECUTIVE SUMMARY 2 1. AN INTRODUCTION TO THE PLASTIC POLLUTION MOVEMENT 4 What are microplastics, microparticles, and microfibers? 6 Why are microplastics a potential threat? 8 What are the pathways and sources of microplastics? 10 2. USING SCIENCE TO GUIDE ACTION 13 Development of this report 14 Use of this report 14 3. SAN FRANCISCO BAY MICROPLASTICS PROJECT FINDINGS 15 Rainy weather washes microplastics into Bay waters 15 Sediment in the lower south Bay had more microplastics 16 Prey fish consume fibers 18 High levels of rubbery fragments and other microplastics and microfibers found in stormwater 18 Wastewater samples dominated by microfibers 19 Quality control samples reveal microfibers are everywhere 20 Related Bay Area microplastics research 20 4. RECOMMENDATIONS FOR SAN FRANCISCO BAY 21 Recommendation #1: Support legislation that reduces single-use plastics and plastic packaging in the Bay Area and statewide 22 Recommendation #2: Explore green stormwater infrastructure management options to reduce microplastics from entering San Francisco Bay 24 Recommendation #3: Identify and prioritize intervention points for microfibers around filtration 26 Recommendation #4: Support the development of a San Francisco Bay Microplastics Management Strategy to reduce microplastics 28 Recommendation #5: Encourage textile industry to standardize methods to understand microfiber -
Study Report Microbeads! Unfold Health Risk and Environmental Pollutant
Study Report Microbeads! Unfold Health Risk and Environmental Pollutant Study Report: Microbeads! Unfold Health Risk and Environmental Pollutant- Copyright © ESDO,2016 BD | Page 2 Study Report: Microbeads! Unfold Health Risk and Environmental Pollutant- Copyright © ESDO,2016 BD | Page 3 Study Report Microbeads! Unfold Health Risk and Environmental Pollutant Advisor Syed Marghub Murshed, Prof. Dr. Abu Jafar Mahmud, Prof. Dr. Md. Abul Hashem, Dr. Shahriar Hossain Study Advisory Team Syed Marghub Murshed, Dr. Shahriar Hossain . Team Leader Siddika Sultana Editorial Team Farida Shahnaz, Sayda Mehrabin Shejuti. Research Associate Nishat Ferdousi Support Team Md. Ali Hossain, Md. Mamun Ul Hasan, Sayda Mehrabin Shejuti , Md. Asif Iqbal. Study Period January2015- October, 2016. Report Published October 15, 2016 Copyright © Environment and Social Development Organization-ESDO, 2016, all rights reserved Disclaimer "While this study and publication have been produced under the organization core funding and direct supervision of research and management team of ESDO, the contents of the publication are the sole responsibility of ESDO. In addition, ESDO has core focussed on plastic pollution and marine littering with the overall objectives of raising public awareness, strengthing capaciity of institutional reserch, policy advocacy to reduce and eliminate the microbeads/microplastics to protect public health and environment in Bangladesh." Contact Address: House # 8/1, Level # 5, Block # C, Lalmatia, Dhaka-1207, Bangladesh, Phone: 880-2-912-2729 Fax: -
Progressing the Microbead Phase Out: Beadrecede Campaign
Progressing the microbead phase out: BeadRecede campaign Craig Brock BSc DipEnvSt MPA Policy & Public Affairs Director Accord Australasia Presentation outline: 1) What is being done to effectively remove plastic microbeads in Australia? 2) What are the advantages of the voluntary industry-led approach which ministers have approved locally? 3) Have their been any findings which differ from the approaches being used in other parts of the world? 4) Are we on track to complete the phase out by the timelines specified? Accord – Who are we? Accord Innovation Strategy: Promote the opportunities for The national industry body in Australia for technology and science to enhance the hygiene, cosmetic and specialty the reputation of the industry as an products industry. innovative solutions provider. Our 100-plus member companies make and/or Our Sustainability Charter is a key part of market the full-range of formulated products used this Innovation Strategy. Environmental in personal care, homecare, industry, institutions stewardship is a key platform of this. and agriculture: cleaning/hygiene products, disinfectants, sanitisers, cosmetics, sunscreens, oral hygiene products, perfumes, adhesives, Environmental stewardship: household pesticides, sealants and protectants: Encouraging a voluntary, progressive • 54% operate in the consumer product market reduction in environmental impact • 41% in the industrial/institutional market throughout the lifecycle of our products by • 5% are associates offering services to industry innovative solutions and reduction -
Technical Review of Microbeads/Microplastics in the Chesapeake Bay
Technical Review of Microbeads/Microplastics in the Chesapeake Bay STAC Review Report Winter 2016 STAC Publication 16-002 About the Scientific and Technical Advisory Committee The Scientific and Technical Advisory Committee (STAC) provides scientific and technical guidance to the Chesapeake Bay Program (CBP) on measures to restore and protect the Chesapeake Bay. Since its creation in December 1984, STAC has worked to enhance scientific communication and outreach throughout the Chesapeake Bay Watershed and beyond. STAC provides scientific and technical advice in various ways, including (1) technical reports and papers, (2) discussion groups, (3) assistance in organizing merit reviews of CBP programs and projects, (4) technical workshops, and (5) interaction between STAC members and the CBP. Through professional and academic contacts and organizational networks of its members, STAC ensures close cooperation among and between the various research institutions and management agencies represented in the Watershed. For additional information about STAC, please visit the STAC website at www.chesapeake.org/stac. Publication Date: April 18, 2016 Publication Number: 16-002 Suggested Citation: Wardrop, D., C. Bott, C. Criddle, R. Hale, J. McDevitt, M. Morse, C. Rochman. 2016. Technical Review of Microbeads/Microplastics in the Chesapeake Bay. STAC Publication Number 16-002, Edgewater, MD. 27 pp. Cover graphic from: Julie Lawson of Trash Free Maryland Mention of trade names or commercial products does not constitute endorsement or recommendation for use. The enclosed material represents the professional recommendations and expert opinion of individuals undertaking a workshop, review, forum, conference, or other activity on a topic or theme that STAC considered an important issue to the goals of the CBP. -
OLA Bulletin Electronically
The Oneida Lake Bulletin Fall 2016 www.oneidalakeassociation.org The American Fisheries Society Publishes a Book on Oneida Lake Oneida Lake: Long-Term Dynamics of a Managed Ecosystem and its Fishery The Oneida Lake Association contin- phosphorous in detergents, expanded ues to explore and discuss the relation- municipal sewer systems, improved ships of physical, chemical, and biotic septic designs, and new storm water ero- components of the lake that contribute sion management has lead to a dramatic to algal blooms. In this column, we are reduction in phosphorous concentrations referencing a new book, recently pub- in Oneida Lake. This drift toward oligo- lished, titled Oneida Lake: Long-Term trophication, however, presents a new Dynamics of a Managed Ecosystem and scenario for Oneida Lake, the decreas- its Fishery (American Fisheries Soci- ing levels of algal carbon. Algal carbon ety 2016), as edited by a provides the nutrition Copepods (photo private-scuba.com) number of our associates for tiny crustacean zoo- at Cornell, including OLA plankton like Daphnia quagga mussels, for example). Board member, Edward and copepods on which Although light penetration has in- Mills. Dr. John Magnu- yellow perch and wall- creased in recent years (leading to pho- son, Professor Emeritus, eye feed in their early tosynthetic activity deeper into the water Center for Limnology, life history. Algal carbon column), such enhanced water clarity has University of Wisconsin, can be reflected in some not translated into more available food Madison acknowledged species that are highly for zooplankton that support juvenile the new book focused on nutritious for Daphnia fishes like perch, walleye, gizzard shad, Oneida Lake as “A Rare while other algae species and buckeye on which the larger fish Book about an Important like blue-greens are es- prey. -
Tecminutesfeb16
O H I O R I V E R V A L L E Y W A T E R S A N I T A T I O N C O M M I S S I O N MINUTES 210th Meeting of the Technical Committee Embassy Suites RiverCenter Covington, KY February 9-10, 2016 Chairman Mike Wilson, Presiding Call to Order The 210th meeting of the ORSANCO Technical Committee was called to order by Chairman Wilson at 1:00 pm on Tuesday, February 9, 2016. Five states, three federal agencies, and three Commission advisory committees were represented (for Roster of Attendance see page 13). Minutes of 209th Committee Meeting ACTION: Motion passed to accept the minutes of the 209th Technical Committee meeting. Chief Engineer’s Report Director Harrison reported that the USEPA released the Toxic Release Inventory on January 21, 2016. The total number of pounds of chemicals released to the Ohio River for 2014 was slightly less than during 2013. He reported that staff is working to develop a five-year projection of finances for the Program and Finance Committee as well as a set of needed projects that are currently unfunded. Finally, he congratulated Ryan Argo and the biological staff on the Montgomery Pool biological report which was made available at the meeting. Overview of ORSANCO’s Technical Programs for the Development of FY17 Recommendations Staff provided an overview of ORSANCO’s current technical programs to facilitate Technical Committee input regarding recommendations for the FY17 program plan. Programs overviewed included water quality monitoring and assessment, biological, pollution control standards, source water protection, and special projects. -
Microbeads: “Tip of the Toxic Plastic-Berg”? Regulation, Alternatives, and Future Implications
Collaborative Masters Program in Science, Society and Policy ISP 5903 – Capstone Practicum Literature Review Microbeads: “Tip of the Toxic Plastic-berg”? Regulation, Alternatives, and Future Implications Submitted to the United States Embassy of Ottawa Brought to the attention of Ms. Rachel So Environment, Water and Fisheries Specialist Nicholas Girard Simon Lester Acacia Paton-Young Dr. Marc Saner (Instructor) April 11, 2016 1 – Table of Contents 2 – Executive Summary .......................................................................................................................................... 3 3 – Timeline ................................................................................................................................................................. 5 4 – Glossary ................................................................................................................................................................. 6 5 – Introduction ......................................................................................................................................................... 7 5.1 Background .................................................................................................................................................. 7 5.2 Our Charge .................................................................................................................................................... 9 6 – Methods ............................................................................................................................................................. -
Microplastic Pollution in the Great Lakes: State, Federal, and Common Law Solutions
ARTICLE Microplastic Pollution in the Great Lakes: State, Federal, and Common Law Solutions NICHOLAS J. SCHROECK* The world’s waterbodies have experienced the effects of plastic pollution for many years. Shorelines and beaches around the world have long been littered with plastic trash. In recent years, the development of large garbage patches in the world’s oceans, including the Eastern Pacific Ocean garbage patch, which has received extensive media coverage,1 and another somewhat smaller one in the Atlantic,2 have grabbed our collective attention. Scientists and researchers have recently shifted their focus to the Great Lakes to determine the extent of plastic pollution in the world’s largest source of fresh water. The first major scientific paper on Great Lakes plastic pollution was published in the Marine Pollution Bulletin, and the results are deeply troubling. The study, a collaborative effort between the 5 Gyres Institute and State University of New York Fredonia, revealed high concentrations of plastics, particularly microplastics, in the Great Lakes.3 Microplastics are those plastics that are less than one millimeter in diameter.4 Microplastics can be separated into two categories. The first category constitutes plastics that are less than five millimeters long and come from * © 2015, Nicholas J. Schroeck. Assistant Clinical Professor, Director of Transnational Environmental Law Clinic, Wayne State University Law School. Thank you to Justin Sterk, Wayne State University Law School (May 2016) for his excellent research assistance. And special thanks to the students at the University of Detroit Mercy Law Review for putting on a wonderful symposium on the Public Trust Doctrine and Great Lakes hazards. -
SIDR: Simultaneous Isolation and Parallel Sequencing of Genomic DNA and Total RNA from Single Cells
Downloaded from genome.cshlp.org on October 3, 2021 - Published by Cold Spring Harbor Laboratory Press 1 SIDR: simultaneous isolation and parallel sequencing of genomic DNA and 2 total RNA from single cells 3 4 Kyung Yeon Han1,9, Kyu-Tae Kim1,9, Je-Gun Joung1,9, Dae-Soon Son1, Yeon Jeong Kim1, Areum Jo1, 5 Hyo-Jeong Jeon1, Hui-Sung Moon1, Chang Eun Yoo1, Woosung Chung1,2, Hye Hyeon Eum1,3, 6 Sangmin Kim4, Hong Kwan Kim5, Jeong Eon Lee2,6, Myung-Ju Ahn7, Hae-Ock Lee1,8, Donghyun 7 Park1,**, Woong-Yang Park1,2,8,* 8 1 Samsung Genome Institute, Samsung Medical Center, Seoul 06351, South Korea 9 2 Department of Health Sciences and Technology, SAIHST, Sungkyunkwan University, Seoul 06351, 10 South Korea 11 3 Department of Biomedical Sciences, College of Medicine, Seoul National University, Seoul 03080, 12 South Korea 13 4 Department of Breast Cancer Center, Samsung Medical Center, Seoul 06351, South Korea 14 5 Department of Thoracic and Cardiovascular Surgery, Samsung Medical Center, Seoul 06351, South 15 Korea 16 6 Department of Surgery, Samsung Medical Center, Sungkyunkwan University School of Medicine, 17 Seoul, 06351, South Korea 18 7 Division of Hematology-Oncology, Department of Medicine, Samsung Medical Center, 19 Sungkyunkwan University School of Medicine, Seoul 06351, South Korea 20 8 Department of Molecular Cell Biology, Sungkyunkwan University School of Medicine, Suwon 16419, 21 South Korea 22 23 * Corresponding author. Tel.: +82 2 2148 9810; fax: +82 2 2148 9819. 24 ** Corresponding author. Tel.: +82 2 3410 2954; fax: +82 2 2148 9819. 25 E-mail addresses: [email protected] (D.