Soft Polyelectrolyte Hydrogel As Versatile Material in Different Application
Total Page:16
File Type:pdf, Size:1020Kb
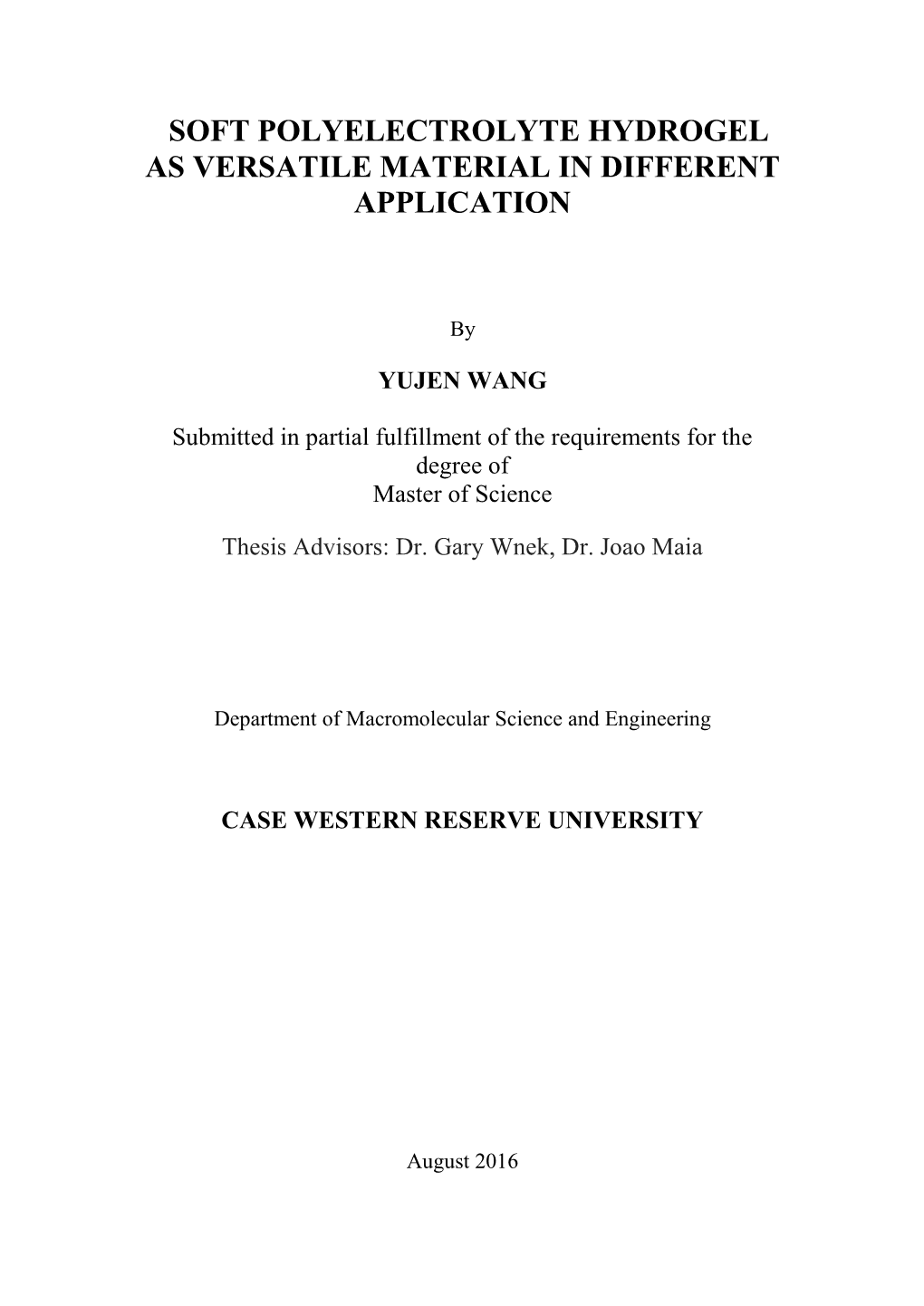
Load more
Recommended publications
-
The Role and Fate of Added Phosphates in Salted Cod Products
The role and fate of added phosphates in salted cod products Kristín Anna Þórarinsdóttir Sigurjón Arason Guðjón Þorkelsson Nýsköpun og neytendur Skýrsla Matís 27-10 Júlí 2010 ISSN 1670-7192 Titill / Title The role and fate of added phosphates in salted cod products / Hlutverk og afdrif viðbætts fosfats í saltfiski Höfundar / Authors Kristín Anna Þórarinsdóttir, Sigurjón Arason, Guðjón Þorkelsson Skýrsla / Report no. 27‐10 Útgáfudagur / Date: Júlí 2010 Verknr. / project no. 1008‐1934, 1008‐1935 Styrktaraðilar / funding: AGS, AVS Ágrip á íslensku: Markmið verkefnisins var að meta afdrif viðbætts fosfats í saltfiski. Ljóst er að magn þess lækkar við verkun og útvötnun. Sama gildir um fosföt sem eru náttúrulega til staðar í fiskvöðva. Þess vegna er heildarmagn fosfats í útvötnuðum afurðum yfirleitt lægra en í ferskum fiski. Hins vegar hefur verið sýnt fram á að viðbætt fosföt (dí‐ og trífosföt) finnast bæði í verkuðum og útvötnum fiski. Það er þó háð magni viðbætts fosfats í afurðinni og hvaða söltunarferlum er beitt, þ.e. hvort fosfati var bætt í fiskinn með sprautun eða pæklun. Lítið eða ekkert greinist í útvötnuðum afurðum ef pæklun er beitt. Munur á milli ferla getur stafað af söltunaraðferð (sprautun/pæklun), gerð og upphaflegu magni viðbætts fosfats og verkunartíma. Frekari rannsókna er þörf til að meta áhrif af mismunandi söltunarferlum á afdrif fosfats í söltuðum þorskvöðva. Lykilorð á íslensku: Saltfiskur, viðbætt fosfat, niðurbrot, verkunarferlar, sprautun, pæklun Summary in English: The aim of this study was to investigate the fate of added phosphates in salted cod products. The content of both added phosphates and naturally occurring phosphates, decreases during salting and rehydration. -
Surface Polarization Effects in Confined Polyelectrolyte Solutions
Surface polarization effects in confined polyelectrolyte solutions Debarshee Bagchia , Trung Dac Nguyenb , and Monica Olvera de la Cruza,b,c,1 aDepartment of Materials Science and Engineering, Northwestern University, Evanston, IL 60208; bDepartment of Chemical and Biological Engineering, Northwestern University, Evanston, IL 60208; and cDepartment of Physics and Astronomy, Northwestern University, Evanston, IL 60208 Contributed by Monica Olvera de la Cruz, June 24, 2020 (sent for review April 21, 2020; reviewed by Rene Messina and Jian Qin) Understanding nanoscale interactions at the interface between ary conditions (18, 19). However, for many biological settings two media with different dielectric constants is crucial for con- as well as in supercapacitor applications, molecular electrolytes trolling many environmental and biological processes, and for confined by dielectric materials, such as graphene, are of interest. improving the efficiency of energy storage devices. In this Recent studies on dielectric confinement of polyelectrolyte by a contributed paper, we show that polarization effects due to spherical cavity showed that dielectric mismatch leads to unex- such dielectric mismatch remarkably influence the double-layer pected symmetry-breaking conformations, as the surface charge structure of a polyelectrolyte solution confined between two density increases (20). The focus of the present study is the col- charged surfaces. Surprisingly, the electrostatic potential across lective effects of spatial confinement by two parallel surfaces -
Assembling of Prussian Blue Nanoclusters Along Single
Assembling of Prussian Blue Nanoclusters Samples preparation. PMB was deposited onto the substrate from the 0.0005 g/l acid water (pH 2, HCl, Aldrich) solution by the dipping of the Along Single Polyelectrolyte Molecules substrate into the solution or by drop casting. To deposit PC chains in stretched conformation we placed several drops of the solution onto Anton Kiriy1, Vera Bocharova1, Ganna Gorodyska1, Sergiy Minko2, the substrate rotating at 10000 rpm. The dry samples were and Manfred Stamm1 investigated with AFM. Deposition of PB clusters. To prepare a dispersion of PB clusters, the 1 Institut für Polymerforschung Dresden, Hohe Strasse 6, 01069 solution of K4Fe(CN)6·3H20 (0.5 g/l, 1.18 mMol/l) in acid water (HCl, pH Dresden, Germany 2.0) and equal volume of the FeCl3 solution (0.048 g/l, 0.296 mMol/l) in 2Department of Chemistry, Clarkson University, Potsdam, NY 13699- acid water (HCl, pH 2.0) were intensively mixed for several minutes. 5810 The substrate with deposited PC was then dipped into the freshly prepared dispersion of PB clusters for 3 min at ambient temperature and afterward rinsed in water. Finally, the substrate was dried with the INTRODUCTION Argon flux. Molecular electronics is attracting considerable interest of AFM measurements. Multimode AFM instrument or NanoScope IV- scientists because of physical and economic limitations expected for D3100 (Digital Instruments, Santa Barbara) were operating in the existing bottom down lithographic technologies. The use of various tapping mode. Silicon tips with radius of 10-20 nm, spring constant of biological templates to assemble nanoscale nonbiological building 30 N/m and resonance frequency of 250-300 KHz were used after the blocks into well-defined meso- and macroscopic objects1 is nn calibration with gold nanoparticles (5 nm in diameter). -
Studies on Metal Complex Formation of Environmentally Friendly Aminopolycarboxylate Chelating Agents
View metadata, citation and similar papers at core.ac.uk brought to you by CORE provided by Helsingin yliopiston digitaalinen arkisto Studies on metal complex formation of environmentally friendly aminopolycarboxylate chelating agents Helena Hyvönen Laboratory of Inorganic Chemistry Department of Chemistry Faculty of Science University of Helsinki Finland Academic dissertation To be presented with the permission of the Faculty of Science of the University of Helsinki for public criticism in Auditorium A110 of the Department of Chemistry, A.I. Virtasen aukio 1, on June 25th 2008 at 12 o’clock noon Helsinki 2008 Supervisor Professor Heikki Saarinen Department of Chemistry University of Helsinki Finland Reviewers Professor Konstantin Popov Physical and Colloid Chemistry Department Moscow State University of Food Production Russia Professor Mika Sillanpää Department of Environmental Sciences University of Kuopio Finland Opponent Professor Lauri Lajunen Department of Chemistry University of Oulu Finland © Helena Hyvönen ISBN 978-952-92-4005-0 (paperback) ISBN 978-952-10-4741-1 (PDF) http://ethesis.helsinki.fi Yliopistopaino Helsinki 2008 2 Abstract For decades, ethylenediaminetetraacetic acid (EDTA) and other aminopolycarboxylates with similar complexation properties and applicability have been widely used as chelating agents in various branches of industry. Recently, the low biodegradability of these ligands and their accumulation in the environment has become cause for concern, because of the persistence of these ligands and their metal complexes in nature. Ethylenediaminedisuccinic acid (EDDS), iminodisuccinic acid (ISA), N-bis[2-(1,2- dicarboxyethoxy)ethyl]aspartic acid (BCA6), N-bis[2-(1,2-dicarboxyethoxy)ethyl]- glycine (BCA5), N-bis[2-(1,2-dicarboxyethoxy)ethyl]methylglycine (MBCA5) and N- tris[(1,2-dicarboxy-ethoxy)ethyl]amine (TCA6) are more environmentally benign and potential candidates to replace EDTA, and also diethylenetriaminepentaacetic acid (DTPA), in several applications. -
S1508 2.5 KG Sodium Tripolyphosphate
Scientific Documentation S1508, Sodium Tripolyphosphate, FCC Not appropriate for regulatory submission. Please visit www.spectrumchemical.com or contact Tech Services for the most up‐to‐date information contained in this information package. Spectrum Chemical Mfg Corp 769 Jersey Avenue New Brunswick, NJ 08901 Phone 732.214.1300 Ver4.01 27.April.2016 Dear Customer, Thank you for your interest in Spectrum’s quality products and services. Spectrum has been proudly serving our scientific community for over 45 years. It is our mission to manufacture and distribute fine chemicals and laboratory products with Quality and delivery you can count on every time. To accomplish our mission, Spectrum utilizes our sourcing leverage and supplier qualification expertise in offering one of the industry’s most comprehensive line of fine chemical products under one brand, in packaging configurations designed to meet your research and production requirements. Our product grades include: USP, NF, BP, EP, JP, FCC, ACS, KSA, Reagent grade, as well as DEA controlled substances. We operate facilities in the United States on the East Coast, West Coast, as well as in Shanghai, China in order to provide the best logistical support for our customers. At Spectrum, Quality is priority number one. Suppliers with the best qualifications are preferred and we employ full-functioning in-house analytical laboratories at each of our facilities. Our facilities and systems are USFDA registered and ISO certified. We frequently host customer audits and cherish opportunities for improvements. Quality is engrained into our culture. Quality is priority number one. In the following pages, we have designed and prepared documented scientific information to aid you in your initial qualification or your continual use of our products. -
Gero Decher, Jean-Claude Voegel La Recherche, No
An Introduction to Polyelectrolyte Multilayers Layer-by-Layer Adsorption (LbL): An Enabling Technology for the Nano- construction of Multifunctional Films on Solvent Accessible Surfaces. G. Decher / Institut Charles Sadron Institut Charles Sadron 1 Differences between chemistry in bulk and at interfaces Some trivia: • Surface functional groups accessible only from the solution side. ( SN1 might be favored over SN2 ; reactivities different from bulk) • Typical monolayer thicknesses of 0.5 nm to 5 nm. • Typical surface areas of 0.20 nm2 per molecule, 5 1014 molecules per cm2. • At a mass of 400 g/mol, 1 cm2 of a densely packed monolayer corresponds to 0.33 µg of material. • 5g (semi-preparative scale), would cover an area of 1500 m2. • Monomolecular layers of polymer may be thinner and less dense and typically consist of 0.1 to 1.5 mg of material per 1 m2. • Less than 0.02 mg for chemical analysis and physical characterization Advantage: We only need tiny amounts from colleagues doing synthesis Institut Charles Sadron 4 Build-to-Order Assembled Films Build-to-Order (BTO) is the capability to quickly build standard or mass-customized products upon receipt of spontaneous orders without forecasts. Layer-by-Layer assembly allows to design functional surfaces and surface-based nano-devices in a "build-to-order" fashion. It exceeds simple self-organization under equilibrium conditions by making it possible to arrange many different materials at will with nanoscale precision. Institut Charles Sadron 5 The multilayer films that can do everything . Pierre Schaaf, Gero Decher, Jean-Claude Voegel La Recherche, No. 389, SEPT. -
UCLA Electronic Theses and Dissertations
UCLA UCLA Electronic Theses and Dissertations Title A Fundamental Perspective on Polyelectrolyte Coagulants and Flocculants in Water Treatment Permalink https://escholarship.org/uc/item/5f30h7k4 Author Bhattacharya, Arkadeep Publication Date 2021 Peer reviewed|Thesis/dissertation eScholarship.org Powered by the California Digital Library University of California UNIVERSITY OF CALIFORNIA Los Angeles A Fundamental Perspective on Polyelectrolyte Coagulants and Flocculants in Water Treatment A thesis submitted in partial satisfaction of the requirements for the degree Master of Science in Chemical Engineering by Arkadeep Bhattacharya 2021 ABSTRACT OF THE THESIS A Fundamental Perspective on Polyelectrolyte Coagulants and Flocculants in Water Treatment by Arkadeep Bhattacharya Master of Science in Chemical Engineering University of California Los Angeles, 2021 Professor Samanvaya Srivastava, Chair Coagulation and flocculation are important phenomena which find widespread applications in water treatment. Polyelectrolytes are charged macromolecules which have found relevance in this domain due to their proven efficiency and effectiveness. The objective of the thesis would be to review and emphasize the fundamental mechanisms on which both natural and synthetic polyelectrolyte coagulants and flocculants operate. Advances in understanding phase characteristics and structure of aggregated polyelectrolyte complexes post interaction with charged impurities are discussed. These would help elucidate the correlation between salient polyelectrolyte properties -
Dispersed and Deposited Polyelectrolyte Complexes and Their Interactions to Chiral Compounds and Proteins
Dispersed and deposited polyelectrolyte complexes and their interactions to chiral compounds and proteins Dissertation zur Erlangung des akademischen Grades Doctor rerum naturalium (Dr. rer. nat.) vorgelegt der Fakultät Mathematik und Naturwissenschaften der Technischen Universität Dresden von M. Sc. Wuye Ouyang geboren am 01.05.1979 in Zhenjiang, V.R. China Gutachter : Prof. Dr. Brigitte Voit Prof. Dr. Thomas Wolff Prof. Dr. Klaus D. Jandt Eingereicht am : 10.10.2008 Tag der Verteidigung: 14.01.2009 ABBREVIATION AFM Atomic force microscopy ASC Ascorbic acid ATR-FTIR Attenuated Total Reflectance - Fourier Transform Infrared Spectroscopy CD Circular dichroism COAC Coacervate phase CSA Camphorsulfonic acid CSP Chiral stationary phases D2O Heavy water / Deuterium oxide DLS Dynamic light scattering DMF Dimethylformamide EtOH Ethanol GA Glutardialdehyde GLU Glutamic acid H2SO4 Sulfuric acid H2O2 Hydrogen peroxide HCl Hydrochloric acid HSA Human serum albumin IEP Isoelectric point IR Infrared IRE Internal reflection element LB Langmuir-Blodgett film LBL Layer by layer LYZ Lysozyme MYO Myoglobin NaCl Sodium chloride NaClO4 Sodium perchlorate NaOH Sodium hydroxide i NC Nitrocellulose PANT Pantothenic acid PBS Phosphate buffer saline PCD Particle charge detector PDADMAC Poly(diallyldimethylammonium chloride) PDL Poly(D-lysine) PDI Polydispersity index PEC Polyelectrolyte complex nanoparticle PEC-0.66 Positively charged polyelectrolyte complex nanoparticle (n-/n+ = 0.66) PEC-1.50 Negatively charged polyelectrolyte complex nanoparticle (n-/n+ = -
(12) United States Patent (10) Patent No.: US 9,023,145 B2 Galembeck Et Al
USOO90231.45B2 (12) United States Patent (10) Patent No.: US 9,023,145 B2 Galembeck et al. (45) Date of Patent: May 5, 2015 (54) ALUMINUM PHOSPHATEOR (58) Field of Classification Search POLYPHOSPHATE COMPOSITIONS USPC .................................. 106/31.13, 287.17, 401 See application file for complete search history. (75) Inventors: Fernando Galembeck, Campinas (BR): Cesar Augusto Sales Barbosa, (56) References Cited Campinas (BR); Melissa Braga, Campinas (BR) U.S. PATENT DOCUMENTS (73) Assignee: Bunge Amorphic Solutions LLC, White 1,654.404 A 12/1927 Blumenberg, Jr. Plains, NY (US) 2,222, 198 A * 1 1/1940 Fleck ......................... 162,1812 (Continued) (*) Notice: Subject to any disclaimer, the term of this patent is extended or adjusted under 35 FOREIGN PATENT DOCUMENTS U.S.C. 154(b) by 730 days. BR IP 9500.522-6 6, 2002 (21) Appl. No.: 12/368,867 BR IP 94.00746-2 T 2003 (Continued) (22) Filed: Feb. 10, 2009 OTHER PUBLICATIONS (65) Prior Publication Data Chemical Book. CAS DataBase List. "Sodium Polyphosphate” US 2009/0217841 A1 Sep. 3, 2009 (2010). http://www.chemicalbook.com/ChemicalProductProperty EN CB0278207.htm.* Related U.S. Application Data (Continued) (60) Provisional application No. 61/065,493, filed on Feb. 12, 2008. Primary Examiner — Kaj K Olsen (51) Int. Cl. Assistant Examiner — Ross J Christie C4B I4/0 (2006.01) (74) Attorney, Agent, or Firm — Snell & Wilmer L.L.P. C09C I/40 (2006.01) B82/30/00 (2011.01) (57) ABSTRACT COIB 25/24025/36 (2006.01)2006.O1 Slurry composition comprising amorphous aluminum phos CSK 3/32 (2OO 6. O R phate, polyphosphate orthophosphate, metaphosphate and/or C09D 5/02 (2OO 6. -
Polyelectrolyte Complex: a Pharmaceutical Review
Review Article Polyelectrolyte Complex: A Pharmaceutical Review Dakhara SL, Anajwala CC Department of Pharmaceutics, Bhagwan Mahavir College of Pharmacy, Surat - 395 017, Gujarat, India ar T ic L E I NF O A bs T rac T Article history: This review work gives a lot of information on polyelectrolyte complexes (PECs). The complex Received 21 April 2010 formed is generally applied in different dosage forms for the formulation of stable aggregated Accepted 2 May 2010 macromolecules. Many properties like diffusion coefficient, chain conformation, viscosity, Available online 07 January 2011 polarizability, miscibility, etc., are drastically changed due to the introduction of a polyelectrolyte. Keywords: The formation of PECs is influenced not only by chemical properties like stereochemical fitting, Beads their molecular weight, charge densities, etc. but also by secondary experimental conditions In vitro release like concentration of polyelectrolytes prior to mixing, their mixing ratio, ionic strength of the Polyelectrolyte complex solution, mixing order, etc. The formation of PECs is described in this article and it is divided into Swelling three main classes, i.e., primary complex formation, formation process within intracomplexes and intercomplex aggregation process. There are different types of PECs obtained according to binding agents such as polymers, proteins, surfactants, drugs, etc. Other factors which affect the formation of PECs are also discussed. There are a number of pharmaceutical applications of polyelectrolytes, such as in controlled -
Stability of ,Aqueous a =Al203 Suspensions with Poly(Methacry1ic
J. Am. Cerum. SOC., 71 14) 250-55 (1988) Stability of ,Aqueous a =Al203Suspensions with Poly(methacry1ic acid) Polyelectrolyte JOSEPH CESARANO III* and ILHAN A. AKSAY* Department of Materials Science and Engiineering, College of Engineering, University of Washington, Seattle, Washington 98 195 ALAN BLEIER* Metals and Ceramics Division, Oak Ridge National Laboratory,* Oak Ridge, Tennessee 3783 1 Stability of aqueous a-A1,O3 suspensions with Na+ salt of have a substantial surface charge of the same sign so that irre- poly(methacry1ic acid) (PMAA-Na) polyelectrolyte was studied versible agglomeration is prevented.' In general, ceramic sus- as a function of pH. At a given pH, the transition from the pensions can be stabilized electrostatically, but improvement of the flocculated to the dispersed state corresponded to the ad- suspensions to better meet the requirements necessary for ceramic sorption saturation limit of the powders by the PMAA. As the processing is possible by incorporating polymeric additives. pH was decreased, the adsorption saturation limit increased Industrial experience shows, for instance, that in highly concen- until insolubility and charge neutralizatioin of the PMAA was trated oxide suspensions, problems related to high viscosity, aging, approached. The critical amount of PMAA required to achieve and processing of multiphase systems can be drastically reduced by stability is outlined in a stability map. using polyelectrolytes as dispersants or deflocc~lants.~.~However, in spite of these advantages in using polyelectrolytes to stabilize suspensions, a great deal of misunderstanding exists in the general ceramic community as to the fundamental roles of these polymeric I. Introduction additives. Thus, this investigation was designed to elucidate the OR many applications in ceramic processing it is desirable to mechanisms of polyelectrolyte stabilization and to relate them to F sinter at relatively low temperatures and to obtain fully dense the chemistry of the powder surface and the polymer additive. -
UNIVERSITY of CALIFORNIA Los Angeles Phase Behavior of Particle
UNIVERSITY OF CALIFORNIA Los Angeles Phase Behavior of Particle-Polyelectrolyte Complexes A thesis submitted in partial satisfaction of the requirements for the degree Master of Science in Chemical Engineering by John E. Neilsen 2019 ABSTRACT OF THE THESIS Phase Behavior of Particle-Polyelectrolyte Complexes by John Neilsen Master of Science in Chemical Engineering University of California, Los Angeles, 2019 Professor Samanvaya Srivastava, Chair The phase behavior of particle-polyelectrolyte complexes was systematically studied using a model system comprising oppositely charged silica nanoparticles and poly(allylamine) hydrochloride (PAH) polycations. Phase behaviors of aqueous mixtures of silica nanoparticles and PAH were elucidated over a wide parameter space of particle and polyelectrolyte concentrations as well as solution pH. Trends in phase behaviors were analyzed to create a fundamental understanding of the fundamental properties that govern the complexation of these oppositely charged species. ii The thesis of John Neilsen is approved. Vasilios Manousiouthakis Junyoung O. Park Samanvaya Srivastava, Committee Chair University of California, Los Angeles 2019 iii Contents 1. Introduction……………………………………………………..………………….…….…..….…..…1 1.1 Aqueous Particle-Polyelectrolyte Self-Assemblies…………..…….....………….....….….1 1.2 Biological Significance …………..……………………..…...….…...…......…….…………..2 1.3 Technological Applications…………..………………….……......……………...………....2 2. Background……………………………………………...………………….……………………..……5 2.1 The Voorn-Overbeek Theory……….………………….…….……………….……….……6