A Search for the Standard Model Higgs Boson Produced in Association with Top Quarks
Total Page:16
File Type:pdf, Size:1020Kb
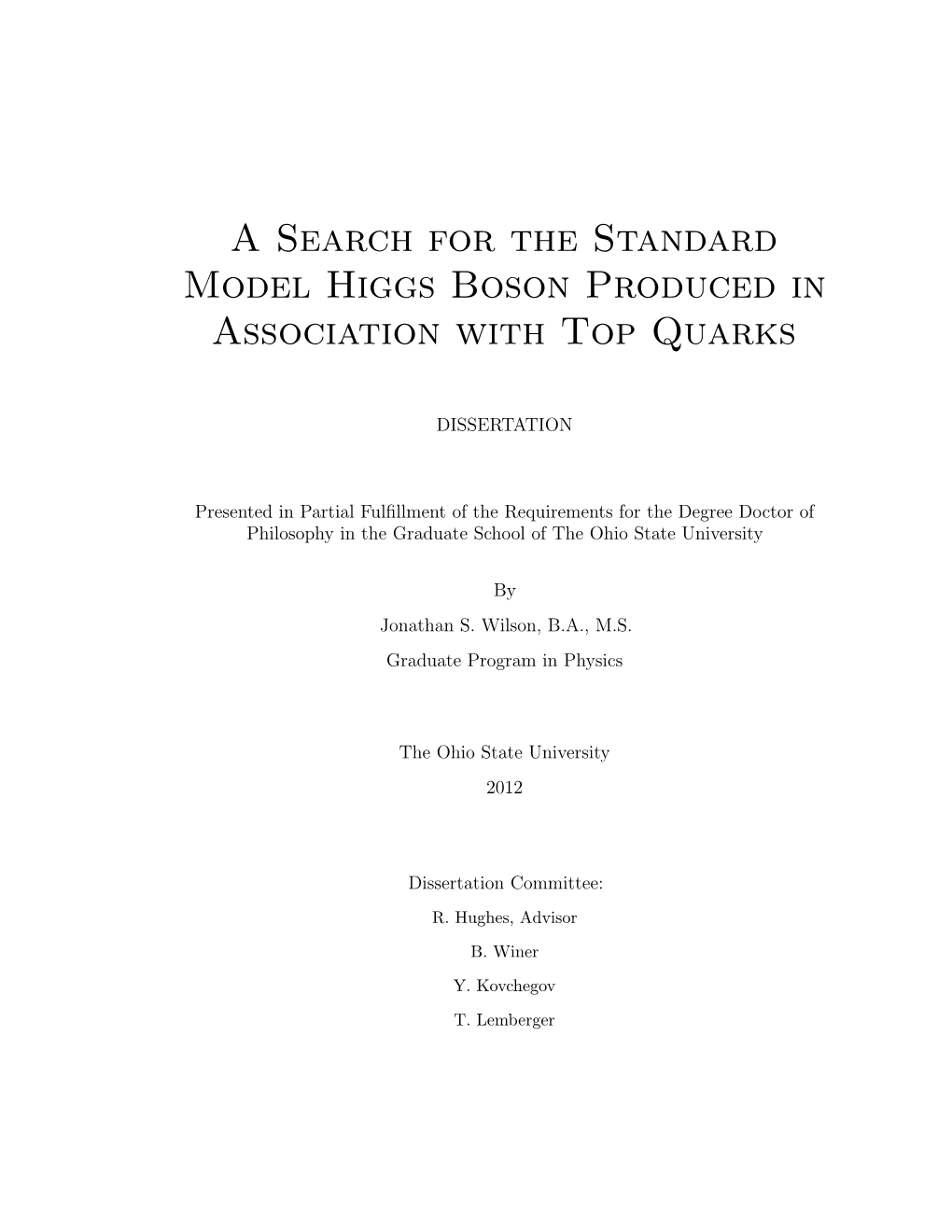
Load more
Recommended publications
-
Higgs Bosons and Supersymmetry
Higgs bosons and Supersymmetry 1. The Higgs mechanism in the Standard Model | The story so far | The SM Higgs boson at the LHC | Problems with the SM Higgs boson 2. Supersymmetry | Surpassing Poincar´e | Supersymmetry motivations | The MSSM 3. Conclusions & Summary D.J. Miller, Edinburgh, July 2, 2004 page 1 of 25 1. Electroweak Symmetry Breaking in the Standard Model 1. Electroweak Symmetry Breaking in the Standard Model Observation: Weak nuclear force mediated by W and Z bosons • M = 80:423 0:039GeV M = 91:1876 0:0021GeV W Z W couples only to left{handed fermions • Fermions have non-zero masses • Theory: We would like to describe electroweak physics by an SU(2) U(1) gauge theory. L ⊗ Y Left{handed fermions are SU(2) doublets Chiral theory ) right{handed fermions are SU(2) singlets f There are two problems with this, both concerning mass: gauge symmetry massless gauge bosons • SU(2) forbids m)( ¯ + ¯ ) terms massless fermions • L L R R L ) D.J. Miller, Edinburgh, July 2, 2004 page 2 of 25 1. Electroweak Symmetry Breaking in the Standard Model Higgs Mechanism Introduce new SU(2) doublet scalar field (φ) with potential V (φ) = λ φ 4 µ2 φ 2 j j − j j Minimum of the potential is not at zero 1 0 µ2 φ = with v = h i p2 v r λ Electroweak symmetry is broken Interactions with scalar field provide: Gauge boson masses • 1 1 2 2 MW = gv MZ = g + g0 v 2 2q Fermion masses • Y ¯ φ m = Y v=p2 f R L −! f f 4 degrees of freedom., 3 become longitudinal components of W and Z, one left over the Higgs boson D.J. -
1 Standard Model: Successes and Problems
Searching for new particles at the Large Hadron Collider James Hirschauer (Fermi National Accelerator Laboratory) Sambamurti Memorial Lecture : August 7, 2017 Our current theory of the most fundamental laws of physics, known as the standard model (SM), works very well to explain many aspects of nature. Most recently, the Higgs boson, predicted to exist in the late 1960s, was discovered by the CMS and ATLAS collaborations at the Large Hadron Collider at CERN in 2012 [1] marking the first observation of the full spectrum of predicted SM particles. Despite the great success of this theory, there are several aspects of nature for which the SM description is completely lacking or unsatisfactory, including the identity of the astronomically observed dark matter and the mass of newly discovered Higgs boson. These and other apparent limitations of the SM motivate the search for new phenomena beyond the SM either directly at the LHC or indirectly with lower energy, high precision experiments. In these proceedings, the successes and some of the shortcomings of the SM are described, followed by a description of the methods and status of the search for new phenomena at the LHC, with some focus on supersymmetry (SUSY) [2], a specific theory of physics beyond the standard model (BSM). 1 Standard model: successes and problems The standard model of particle physics describes the interactions of fundamental matter particles (quarks and leptons) via the fundamental forces (mediated by the force carrying particles: the photon, gluon, and weak bosons). The Higgs boson, also a fundamental SM particle, plays a central role in the mechanism that determines the masses of the photon and weak bosons, as well as the rest of the standard model particles. -
An Introduction to the Quark Model
An Introduction to the Quark Model Garth Huber Prairie Universities Physics Seminar Series, November, 2009. Particles in Atomic Physics • View of the particle world as of early 20th Century. • Particles found in atoms: –Electron – Nucleons: •Proton(nucleus of hydrogen) •Neutron(e.g. nucleus of helium – α-particle - has two protons and two neutrons) • Related particle mediating electromagnetic interactions between electrons and protons: Particle Electric charge Mass – Photon (light!) (x 1.6 10-19 C) (GeV=x 1.86 10-27 kg) e −1 0.0005 p +1 0.938 n 0 0.940 γ 0 0 Dr. Garth Huber, Dept. of Physics, Univ. of Regina, Regina, SK S4S0A2, Canada. 2 Early Evidence for Nucleon Internal Structure • Apply the Correspondence Principle to the Classical relation for q magnetic moment: µ = L 2m • Obtain for a point-like spin-½ particle of mass mp: qqeq⎛⎞⎛⎞ µ == =µ ⎜⎟ ⎜⎟ N 2222memepp⎝⎠ ⎝⎠ 2 Experimental values: µp=2.79 µN (p) µn= -1.91 µN (n) • Experimental values inconsistent with point-like assumption. • In particular, the neutron’s magnetic moment does not vanish, as expected for a point-like electrically neutral particle. This is unequivocal evidence that the neutron (and proton) has an internal structure involving a distribution of charges. Dr. Garth Huber, Dept. of Physics, Univ. of Regina, Regina, SK S4S0A2, Canada. 3 The Particle Zoo • Circa 1950, the first particle accelerators began to uncover many new particles. • Most of these particles are unstable and decay very quickly, and hence had not been seen in cosmic ray experiments. • Could all these particles be fundamental? Dr. Garth Huber, Dept. -
A Young Physicist's Guide to the Higgs Boson
A Young Physicist’s Guide to the Higgs Boson Tel Aviv University Future Scientists – CERN Tour Presented by Stephen Sekula Associate Professor of Experimental Particle Physics SMU, Dallas, TX Programme ● You have a problem in your theory: (why do you need the Higgs Particle?) ● How to Make a Higgs Particle (One-at-a-Time) ● How to See a Higgs Particle (Without fooling yourself too much) ● A View from the Shadows: What are the New Questions? (An Epilogue) Stephen J. Sekula - SMU 2/44 You Have a Problem in Your Theory Credit for the ideas/example in this section goes to Prof. Daniel Stolarski (Carleton University) The Usual Explanation Usual Statement: “You need the Higgs Particle to explain mass.” 2 F=ma F=G m1 m2 /r Most of the mass of matter lies in the nucleus of the atom, and most of the mass of the nucleus arises from “binding energy” - the strength of the force that holds particles together to form nuclei imparts mass-energy to the nucleus (ala E = mc2). Corrected Statement: “You need the Higgs Particle to explain fundamental mass.” (e.g. the electron’s mass) E2=m2 c4+ p2 c2→( p=0)→ E=mc2 Stephen J. Sekula - SMU 4/44 Yes, the Higgs is important for mass, but let’s try this... ● No doubt, the Higgs particle plays a role in fundamental mass (I will come back to this point) ● But, as students who’ve been exposed to introductory physics (mechanics, electricity and magnetism) and some modern physics topics (quantum mechanics and special relativity) you are more familiar with.. -
1 Quark Model of Hadrons
1 QUARK MODEL OF HADRONS 1 Quark model of hadrons 1.1 Symmetries and evidence for quarks 1 Hadrons as bound states of quarks – point-like spin- 2 objects, charged (‘coloured’) under the strong force. Baryons as qqq combinations. Mesons as qq¯ combinations. 1 Baryon number = 3 (N(q) − N(¯q)) and its conservation. You are not expected to know all of the names of the particles in the baryon and meson multiplets, but you are expected to know that such multiplets exist, and to be able to interpret them if presented with them. You should also know the quark contents of the simple light baryons and mesons (and their anti-particles): p = (uud) n = (udd) π0 = (mixture of uu¯ and dd¯) π+ = (ud¯) K0 = (ds¯) K+ = (us¯). and be able to work out others with some hints. P 1 + Lowest-lying baryons as L = 0 and J = 2 states of qqq. P 3 + Excited versions have L = 0 and J = 2 . Pauli exclusion and non existence of uuu, ddd, sss states in lowest lying multiplet. Lowest-lying mesons as qq¯0 states with L = 0 and J P = 0−. First excited levels (particles) with same quark content have L = 0 and J P = 1−. Ability to explain contents of these multiplets in terms of quarks. J/Ψ as a bound state of cc¯ Υ (upsilon) as a bound state of b¯b. Realization that these are hydrogenic-like states with suitable reduced mass (c.f. positronium), and subject to the strong force, so with energy levels paramaterized by αs rather than αEM Top is very heavy and decays before it can form hadrons, so no top hadrons exist. -
The Structure of Quarks and Leptons
The Structure of Quarks and Leptons They have been , considered the elementary particles ofmatter, but instead they may consist of still smaller entities confjned within a volume less than a thousandth the size of a proton by Haim Harari n the past 100 years the search for the the quark model that brought relief. In imagination: they suggest a way of I ultimate constituents of matter has the initial formulation of the model all building a complex world out of a few penetrated four layers of structure. hadrons could be explained as combina simple parts. All matter has been shown to consist of tions of just three kinds of quarks. atoms. The atom itself has been found Now it is the quarks and leptons Any theory of the elementary particles to have a dense nucleus surrounded by a themselves whose proliferation is begin fl. of matter must also take into ac cloud of electrons. The nucleus in turn ning to stir interest in the possibility of a count the forces that act between them has been broken down into its compo simpler-scheme. Whereas the original and the laws of nature that govern the nent protons and neutrons. More recent model had three quarks, there are now forces. Little would be gained in simpli ly it has become apparent that the pro thought to be at least 18, as well as six fying the spectrum of particles if the ton and the neutron are also composite leptons and a dozen other particles that number of forces and laws were thereby particles; they are made up of the small act as carriers of forces. -
New Physics of Strong Interaction and Dark Universe
universe Review New Physics of Strong Interaction and Dark Universe Vitaly Beylin 1 , Maxim Khlopov 1,2,3,* , Vladimir Kuksa 1 and Nikolay Volchanskiy 1,4 1 Institute of Physics, Southern Federal University, Stachki 194, 344090 Rostov on Don, Russia; [email protected] (V.B.); [email protected] (V.K.); [email protected] (N.V.) 2 CNRS, Astroparticule et Cosmologie, Université de Paris, F-75013 Paris, France 3 National Research Nuclear University “MEPHI” (Moscow State Engineering Physics Institute), 31 Kashirskoe Chaussee, 115409 Moscow, Russia 4 Bogoliubov Laboratory of Theoretical Physics, Joint Institute for Nuclear Research, Joliot-Curie 6, 141980 Dubna, Russia * Correspondence: [email protected]; Tel.:+33-676380567 Received: 18 September 2020; Accepted: 21 October 2020; Published: 26 October 2020 Abstract: The history of dark universe physics can be traced from processes in the very early universe to the modern dominance of dark matter and energy. Here, we review the possible nontrivial role of strong interactions in cosmological effects of new physics. In the case of ordinary QCD interaction, the existence of new stable colored particles such as new stable quarks leads to new exotic forms of matter, some of which can be candidates for dark matter. New QCD-like strong interactions lead to new stable composite candidates bound by QCD-like confinement. We put special emphasis on the effects of interaction between new stable hadrons and ordinary matter, formation of anomalous forms of cosmic rays and exotic forms of matter, like stable fractionally charged particles. The possible correlation of these effects with high energy neutrino and cosmic ray signatures opens the way to study new physics of strong interactions by its indirect multi-messenger astrophysical probes. -
Properties of Baryons in the Chiral Quark Model
Properties of Baryons in the Chiral Quark Model Tommy Ohlsson Teknologie licentiatavhandling Kungliga Tekniska Hogskolan¨ Stockholm 1997 Properties of Baryons in the Chiral Quark Model Tommy Ohlsson Licentiate Dissertation Theoretical Physics Department of Physics Royal Institute of Technology Stockholm, Sweden 1997 Typeset in LATEX Akademisk avhandling f¨or teknologie licentiatexamen (TeknL) inom ¨amnesomr˚adet teoretisk fysik. Scientific thesis for the degree of Licentiate of Engineering (Lic Eng) in the subject area of Theoretical Physics. TRITA-FYS-8026 ISSN 0280-316X ISRN KTH/FYS/TEO/R--97/9--SE ISBN 91-7170-211-3 c Tommy Ohlsson 1997 Printed in Sweden by KTH H¨ogskoletryckeriet, Stockholm 1997 Properties of Baryons in the Chiral Quark Model Tommy Ohlsson Teoretisk fysik, Institutionen f¨or fysik, Kungliga Tekniska H¨ogskolan SE-100 44 Stockholm SWEDEN E-mail: [email protected] Abstract In this thesis, several properties of baryons are studied using the chiral quark model. The chiral quark model is a theory which can be used to describe low energy phenomena of baryons. In Paper 1, the chiral quark model is studied using wave functions with configuration mixing. This study is motivated by the fact that the chiral quark model cannot otherwise break the Coleman–Glashow sum-rule for the magnetic moments of the octet baryons, which is experimentally broken by about ten standard deviations. Configuration mixing with quark-diquark components is also able to reproduce the octet baryon magnetic moments very accurately. In Paper 2, the chiral quark model is used to calculate the decuplet baryon ++ magnetic moments. The values for the magnetic moments of the ∆ and Ω− are in good agreement with the experimental results. -
MIT at the Large Hadron Collider—Illuminating the High-Energy Frontier
Mit at the large hadron collider—Illuminating the high-energy frontier 40 ) roland | klute mit physics annual 2010 gunther roland and Markus Klute ver the last few decades, teams of physicists and engineers O all over the globe have worked on the components for one of the most complex machines ever built: the Large Hadron Collider (LHC) at the CERN laboratory in Geneva, Switzerland. Collaborations of thousands of scientists have assembled the giant particle detectors used to examine collisions of protons and nuclei at energies never before achieved in a labo- ratory. After initial tests proved successful in late 2009, the LHC physics program was launched in March 2010. Now the race is on to fulfill the LHC’s paradoxical mission: to complete the Stan- dard Model of particle physics by detecting its last missing piece, the Higgs boson, and to discover the building blocks of a more complete theory of nature to finally replace the Standard Model. The MIT team working on the Compact Muon Solenoid (CMS) experiment at the LHC stands at the forefront of this new era of particle and nuclear physics. The High Energy Frontier Our current understanding of the fundamental interactions of nature is encap- sulated in the Standard Model of particle physics. In this theory, the multitude of subatomic particles is explained in terms of just two kinds of basic building blocks: quarks, which form protons and neutrons, and leptons, including the electron and its heavier cousins. From the three basic interactions described by the Standard Model—the strong, electroweak and gravitational forces—arise much of our understanding of the world around us, from the formation of matter in the early universe, to the energy production in the Sun, and the stability of atoms and mit physics annual 2010 roland | klute ( 41 figure 1 A photograph of the interior, central molecules. -
Introduction to Supersymmetry
Introduction to Supersymmetry Pre-SUSY Summer School Corpus Christi, Texas May 15-18, 2019 Stephen P. Martin Northern Illinois University [email protected] 1 Topics: Why: Motivation for supersymmetry (SUSY) • What: SUSY Lagrangians, SUSY breaking and the Minimal • Supersymmetric Standard Model, superpartner decays Who: Sorry, not covered. • For some more details and a slightly better attempt at proper referencing: A supersymmetry primer, hep-ph/9709356, version 7, January 2016 • TASI 2011 lectures notes: two-component fermion notation and • supersymmetry, arXiv:1205.4076. If you find corrections, please do let me know! 2 Lecture 1: Motivation and Introduction to Supersymmetry Motivation: The Hierarchy Problem • Supermultiplets • Particle content of the Minimal Supersymmetric Standard Model • (MSSM) Need for “soft” breaking of supersymmetry • The Wess-Zumino Model • 3 People have cited many reasons why extensions of the Standard Model might involve supersymmetry (SUSY). Some of them are: A possible cold dark matter particle • A light Higgs boson, M = 125 GeV • h Unification of gauge couplings • Mathematical elegance, beauty • ⋆ “What does that even mean? No such thing!” – Some modern pundits ⋆ “We beg to differ.” – Einstein, Dirac, . However, for me, the single compelling reason is: The Hierarchy Problem • 4 An analogy: Coulomb self-energy correction to the electron’s mass A point-like electron would have an infinite classical electrostatic energy. Instead, suppose the electron is a solid sphere of uniform charge density and radius R. An undergraduate problem gives: 3e2 ∆ECoulomb = 20πǫ0R 2 Interpreting this as a correction ∆me = ∆ECoulomb/c to the electron mass: 15 0.86 10− meters m = m + (1 MeV/c2) × . -
Light Higgs Production in Hyperon Decay
Light Higgs Production in Hyperon Decay Xiao-Gang He∗ Department of Physics and Center for Theoretical Sciences, National Taiwan University, Taipei. Jusak Tandean† Department of Mathematics/Physics/Computer Science, University of La Verne, La Verne, CA 91750, USA G. Valencia‡ Department of Physics and Astronomy, Iowa State University, Ames, IA 50011, USA (Dated: July 8, 2018) Abstract A recent HyperCP observation of three events in the decay Σ+ pµ+µ− is suggestive of a new particle → with mass 214.3 MeV. In order to confront models that contain a light Higgs boson with this observation, it is necessary to know the Higgs production rate in hyperon decay. The contribution to this rate from penguin-like two-quark operators has been considered before and found to be too large. We point out that there are additional four-quark contributions to this rate that could be comparable in size to the two-quark contributions, and that could bring the total rate to the observed level in some models. To this effect we implement the low-energy theorems that dictate the couplings of light Higgs bosons to hyperons at leading order in chiral perturbation theory. We consider the cases of scalar and pseudoscalar Higgs bosons in the standard model and in its two-Higgs-doublet extensions to illustrate the challenges posed by existing experimental constraints and suggest possible avenues for models to satisfy them. arXiv:hep-ph/0610274v3 16 Apr 2008 ∗Electronic address: [email protected] †Electronic address: [email protected] ‡Electronic address: [email protected] 1 I. INTRODUCTION Three events for the decay mode Σ+ pµ+µ− with a dimuon invariant mass of 214.3 0.5 MeV → ± have been recently observed by the HyperCP Collaboration [1]. -
Exotic Quarks in Twin Higgs Models
Prepared for submission to JHEP SLAC-PUB-16433, KIAS-P15042, UMD-PP-015-016 Exotic Quarks in Twin Higgs Models Hsin-Chia Cheng,1 Sunghoon Jung,2;3 Ennio Salvioni,1 and Yuhsin Tsai1;4 1Department of Physics, University of California, Davis, Davis, CA 95616, USA 2Korea Institute for Advanced Study, Seoul 130-722, Korea 3SLAC National Accelerator Laboratory, 2575 Sand Hill Road, Menlo Park, CA 94025, USA 4Maryland Center for Fundamental Physics, Department of Physics, University of Maryland, College Park, MD 20742, USA E-mail: [email protected], [email protected], [email protected], [email protected] Abstract: The Twin Higgs model provides a natural theory for the electroweak symmetry breaking without the need of new particles carrying the standard model gauge charges below a few TeV. In the low energy theory, the only probe comes from the mixing of the Higgs fields in the standard model and twin sectors. However, an ultraviolet completion is required below ∼ 10 TeV to remove residual logarithmic divergences. In non-supersymmetric completions, new exotic fermions charged under both the standard model and twin gauge symmetries have to be present to accompany the top quark, thus providing a high energy probe of the model. Some of them carry standard model color, and may therefore be copiously produced at current or future hadron colliders. Once produced, these exotic quarks can decay into a top together with twin sector particles. If the twin sector particles escape the detection, we have the irreducible stop-like signals. On the other hand, some twin sector particles may decay back into the standard model particles with long lifetimes, giving spectacular displaced vertex signals in combination with the prompt top quarks.