The Unusual Structure of the Ubiquitin-Like Domain of the Protein
Total Page:16
File Type:pdf, Size:1020Kb
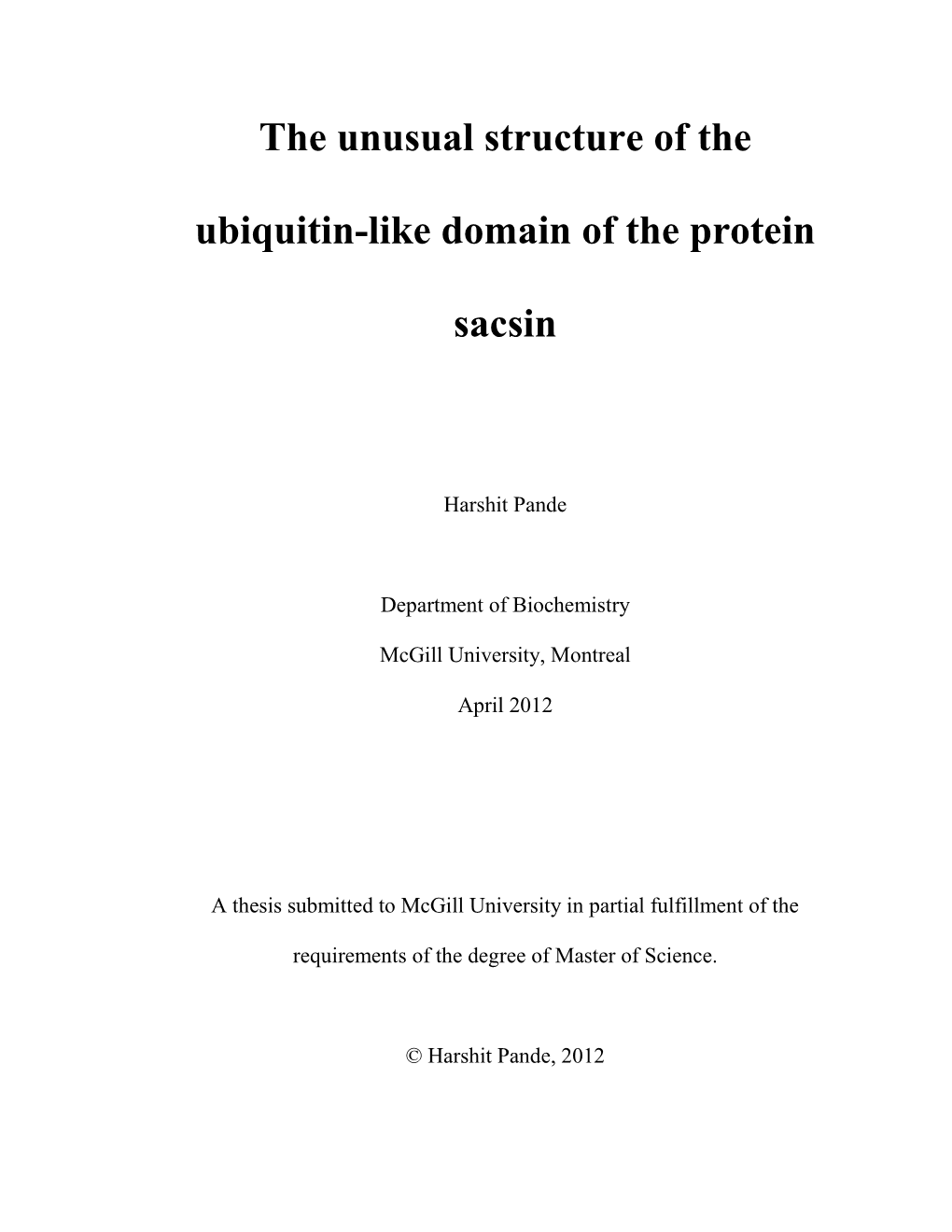
Load more
Recommended publications
-
Environmental Influences on Endothelial Gene Expression
ENDOTHELIAL CELL GENE EXPRESSION John Matthew Jeff Herbert Supervisors: Prof. Roy Bicknell and Dr. Victoria Heath PhD thesis University of Birmingham August 2012 University of Birmingham Research Archive e-theses repository This unpublished thesis/dissertation is copyright of the author and/or third parties. The intellectual property rights of the author or third parties in respect of this work are as defined by The Copyright Designs and Patents Act 1988 or as modified by any successor legislation. Any use made of information contained in this thesis/dissertation must be in accordance with that legislation and must be properly acknowledged. Further distribution or reproduction in any format is prohibited without the permission of the copyright holder. ABSTRACT Tumour angiogenesis is a vital process in the pathology of tumour development and metastasis. Targeting markers of tumour endothelium provide a means of targeted destruction of a tumours oxygen and nutrient supply via destruction of tumour vasculature, which in turn ultimately leads to beneficial consequences to patients. Although current anti -angiogenic and vascular targeting strategies help patients, more potently in combination with chemo therapy, there is still a need for more tumour endothelial marker discoveries as current treatments have cardiovascular and other side effects. For the first time, the analyses of in-vivo biotinylation of an embryonic system is performed to obtain putative vascular targets. Also for the first time, deep sequencing is applied to freshly isolated tumour and normal endothelial cells from lung, colon and bladder tissues for the identification of pan-vascular-targets. Integration of the proteomic, deep sequencing, public cDNA libraries and microarrays, delivers 5,892 putative vascular targets to the science community. -
Apoptosis Induced by Proteasome Inhibition in Cancer Cells: Predominant Role of the P53/PUMA Pathway
Oncogene (2007) 26, 1681–1692 & 2007 Nature Publishing Group All rights reserved 0950-9232/07 $30.00 www.nature.com/onc ORIGINAL ARTICLE Apoptosis induced by proteasome inhibition in cancer cells: predominant role of the p53/PUMA pathway CG Concannon1, BF Koehler1,2, Claus Reimertz2, BM Murphy1, C Bonner1, N Thurow2, MW Ward1, AVillunger 3, AStrasser 4,DKo¨ gel2,5 and JHM Prehn1,5 1Department of Physiology and Medical Physics, Royal College of Surgeons in Ireland, Dublin, Ireland; 2Experimental Neurosurgery, Centre for Neurology and Neurosurgery, Johann Wolfgang Goethe University Clinics, Theodor-Stern-Kai 7, Frankfurt/Main, Germany; 3Division of Experimental Pathophysiology and Immunology, Biocenter, Innsbruck Medical University, Innsbruck, Austria and 4The Walter and Eliza Hall Institute of Medical Research, Melbourne, Australia The proteasome has emerged as a novel target for Introduction antineoplastic treatment of hematological malignancies and solid tumors, including those of the central nervous The correct functioning of the ubiquitin-proteasome system. To identify cell death pathways activated in pathway is essential for the degradation of the majority response to inhibition of the proteasome system in cancer of intracellular proteins. Several key regulatory proteins cells, we treated human SH-SY5Y neuroblastoma cells involved in cell proliferation and differentiation are with the selective proteasome inhibitor (PI) epoxomicin regulated by proteasome-mediated proteolysis resulting (Epoxo). Prolonged exposure to Epoxo was associated in the activation or inhibition of specific cell signaling with increased levels of poly-ubiquitinylated proteins and pathways (Adams, 2004a). The proteasome is also p53, release of cytochrome c from the mitochondria, and central to the regulation of cell death and apoptosis. -
Figure S1. DMD Module Network. the Network Is Formed by 260 Genes from Disgenet and 1101 Interactions from STRING. Red Nodes Are the Five Seed Candidate Genes
Figure S1. DMD module network. The network is formed by 260 genes from DisGeNET and 1101 interactions from STRING. Red nodes are the five seed candidate genes. Figure S2. DMD module network is more connected than a random module of the same size. It is shown the distribution of the largest connected component of 10.000 random modules of the same size of the DMD module network. The green line (x=260) represents the DMD largest connected component, obtaining a z-score=8.9. Figure S3. Shared genes between BMD and DMD signature. A) A meta-analysis of three microarray datasets (GSE3307, GSE13608 and GSE109178) was performed for the identification of differentially expressed genes (DEGs) in BMD muscle biopsies as compared to normal muscle biopsies. Briefly, the GSE13608 dataset included 6 samples of skeletal muscle biopsy from healthy people and 5 samples from BMD patients. Biopsies were taken from either biceps brachii, triceps brachii or deltoid. The GSE3307 dataset included 17 samples of skeletal muscle biopsy from healthy people and 10 samples from BMD patients. The GSE109178 dataset included 14 samples of controls and 11 samples from BMD patients. For both GSE3307 and GSE10917 datasets, biopsies were taken at the time of diagnosis and from the vastus lateralis. For the meta-analysis of GSE13608, GSE3307 and GSE109178, a random effects model of effect size measure was used to integrate gene expression patterns from the two datasets. Genes with an adjusted p value (FDR) < 0.05 and an │effect size│>2 were identified as DEGs and selected for further analysis. A significant number of DEGs (p<0.001) were in common with the DMD signature genes (blue nodes), as determined by a hypergeometric test assessing the significance of the overlap between the BMD DEGs and the number of DMD signature genes B) MCODE analysis of the overlapping genes between BMD DEGs and DMD signature genes. -
A Computational Approach for Defining a Signature of Β-Cell Golgi Stress in Diabetes Mellitus
Page 1 of 781 Diabetes A Computational Approach for Defining a Signature of β-Cell Golgi Stress in Diabetes Mellitus Robert N. Bone1,6,7, Olufunmilola Oyebamiji2, Sayali Talware2, Sharmila Selvaraj2, Preethi Krishnan3,6, Farooq Syed1,6,7, Huanmei Wu2, Carmella Evans-Molina 1,3,4,5,6,7,8* Departments of 1Pediatrics, 3Medicine, 4Anatomy, Cell Biology & Physiology, 5Biochemistry & Molecular Biology, the 6Center for Diabetes & Metabolic Diseases, and the 7Herman B. Wells Center for Pediatric Research, Indiana University School of Medicine, Indianapolis, IN 46202; 2Department of BioHealth Informatics, Indiana University-Purdue University Indianapolis, Indianapolis, IN, 46202; 8Roudebush VA Medical Center, Indianapolis, IN 46202. *Corresponding Author(s): Carmella Evans-Molina, MD, PhD ([email protected]) Indiana University School of Medicine, 635 Barnhill Drive, MS 2031A, Indianapolis, IN 46202, Telephone: (317) 274-4145, Fax (317) 274-4107 Running Title: Golgi Stress Response in Diabetes Word Count: 4358 Number of Figures: 6 Keywords: Golgi apparatus stress, Islets, β cell, Type 1 diabetes, Type 2 diabetes 1 Diabetes Publish Ahead of Print, published online August 20, 2020 Diabetes Page 2 of 781 ABSTRACT The Golgi apparatus (GA) is an important site of insulin processing and granule maturation, but whether GA organelle dysfunction and GA stress are present in the diabetic β-cell has not been tested. We utilized an informatics-based approach to develop a transcriptional signature of β-cell GA stress using existing RNA sequencing and microarray datasets generated using human islets from donors with diabetes and islets where type 1(T1D) and type 2 diabetes (T2D) had been modeled ex vivo. To narrow our results to GA-specific genes, we applied a filter set of 1,030 genes accepted as GA associated. -
A Reduction in Drp1-Mediated Fission Compromises
HMG Advance Access published June 26, 2016 Human Molecular Genetics, 2016, Vol. 0, No. 0 1–13 doi: 10.1093/hmg/ddw173 Advance Access Publication Date: 10 June 2016 Original Article ORIGINAL ARTICLE A reduction in Drp1-mediated fission compromises mitochondrial health in autosomal recessive spastic Downloaded from ataxia of Charlevoix Saguenay Teisha Y. Bradshaw1, Lisa E.L. Romano1, Emma J. Duncan1, 1 3 2 3 Suran Nethisinghe , Rosella Abeti , Gregory J. Michael , Paola Giunti , http://hmg.oxfordjournals.org/ Sascha Vermeer4 and J. Paul Chapple1,* 1William Harvey Research Institute, Barts and the London School of Medicine, Queen Mary University of London, London EC1M 6BQ, United Kingdom, 2Blizard Institute, Barts and the London School of Medicine and Dentistry, Queen Mary University of London, London E1 2AT, United Kingdom, 3Department of Molecular Neuroscience, UCL Institute of Neurology, London WC1N 3BG, United Kingdom and 4Department of Clinical Genetics, The Netherlands Cancer Institute, 1066 CX Amsterdam, The Netherlands at University College London on December 9, 2016 *To whom correspondence should be addressed at: Tel: þ44 2078826242; Fax: þ44 207 882 6197; Email [email protected] Abstract The neurodegenerative disease autosomal recessive spastic ataxia of Charlevoix Saguenay (ARSACS) is caused by loss of function of sacsin, a modular protein that is required for normal mitochondrial network organization. To further understand cellular consequences of loss of sacsin, we performed microarray analyses in sacsin knockdown cells and ARSACS patient fibroblasts. This identified altered transcript levels for oxidative phosphorylation and oxidative stress genes. These changes in mitochondrial gene networks were validated by quantitative reverse transcription PCR. -
Product Description P441-A2 SACS-V01
MRC-Holland ® Product Description version A2-01; Issued 23 April 2020 MLPA Product Description SALSA® MLPA® Probemix P441-A2 SACS To be used with the MLPA General Protocol. Version A2. Compared to version A1, four reference probes have been replaced. For complete product history see page 5. Catalogue numbers: • P441-025R: SALSA MLPA Probemix P441 SACS, 25 reactions. • P441-050R: SALSA MLPA Probemix P441 SACS, 50 reactions. • P441-100R: SALSA MLPA Probemix P441 SACS, 100 reactions. To be used in combination with a SALSA MLPA reagent kit and Coffalyser.Net data analysis software. MLPA reagent kits are either provided with FAM or Cy5.0 dye-labelled PCR primer, suitable for Applied Biosystems and Beckman/SCIEX capillary sequencers, respectively (see www.mlpa.com). Certificate of Analysis: Information regarding storage conditions, quality tests, and a sample electropherogram from the current sales lot is available at www.mlpa.com. Precautions and warnings: For professional use only. Always consult the most recent product description AND the MLPA General Protocol before use: www.mlpa.com. It is the responsibility of the user to be aware of the latest scientific knowledge of the application before drawing any conclusions from findings generated with this product. General information: The SALSA MLPA Probemix P441 SACS is a research use only (RUO) assay for the detection of deletions or duplications in the SACS gene, which is associated with autosomal recessive spastic ataxia of Charlevoix-Saguenay (ARSACS). ARSACS is a neurodegenerative disorder, characterised by early-onset progressive cerebellar ataxia with spasticity and peripheral neuropathy. The classic form of ARSACS is often displayed in early childhood, leading to delayed walking in young toddlers, while individuals with disease onset in teenage or early-adult years are also being described more recently. -
1 Mutational Heterogeneity in Cancer Akash Kumar a Dissertation
Mutational Heterogeneity in Cancer Akash Kumar A dissertation Submitted in partial fulfillment of requirements for the degree of Doctor of Philosophy University of Washington 2014 June 5 Reading Committee: Jay Shendure Pete Nelson Mary Claire King Program Authorized to Offer Degree: Genome Sciences 1 University of Washington ABSTRACT Mutational Heterogeneity in Cancer Akash Kumar Chair of the Supervisory Committee: Associate Professor Jay Shendure Department of Genome Sciences Somatic mutation plays a key role in the formation and progression of cancer. Differences in mutation patterns likely explain much of the heterogeneity seen in prognosis and treatment response among patients. Recent advances in massively parallel sequencing have greatly expanded our capability to investigate somatic mutation. Genomic profiling of tumor biopsies could guide the administration of targeted therapeutics on the basis of the tumor’s collection of mutations. Central to the success of this approach is the general applicability of targeted therapies to a patient’s entire tumor burden. This requires a better understanding of the genomic heterogeneity present both within individual tumors (intratumoral) and amongst tumors from the same patient (intrapatient). My dissertation is broadly organized around investigating mutational heterogeneity in cancer. Three projects are discussed in detail: analysis of (1) interpatient and (2) intrapatient heterogeneity in men with disseminated prostate cancer, and (3) investigation of regional intratumoral heterogeneity in -
Population Genetics of the Coral Acropora Millepora: Towards a Genomic Predictor of Bleaching
bioRxiv preprint doi: https://doi.org/10.1101/867754; this version posted December 6, 2019. The copyright holder for this preprint (which was not certified by peer review) is the author/funder. All rights reserved. No reuse allowed without permission. Population genetics of the coral Acropora millepora: Towards a genomic predictor of bleaching Zachary L. Fuller∗1, Veronique J.L. Mocellin2, Luke Morris2, Neal Cantin2, Jihanne Shepherd1, Luke Sarre1, Julie Peng3, Yi Liao4,5, Joseph Pickrell6, Peter Andolfatto1, Mikhail Matzy4, Line K. Bayy2, and Molly Przeworskiy1,2,3 1Department of Biological Sciences, Columbia University 2Australia Institute of Marine Science 3Lewis-Sigler Institute for Integrative Genomics, Princeton University 4Department of Integrative Biology, University of Texas at Austin 5Department of Ecology and Evolutionary Biology, University of California, Irvine 6Gencove, Inc. New York 7Department of Systems Biology, Columbia University 8Program for Mathematical Genomics, Columbia University 6 December 2019 Abstract Although reef-building corals are rapidly declining worldwide, responses to bleaching vary both within and among species. Because these inter-individual differences are partly her- itable, they should in principle be predictable from genomic data. Towards that goal, we generated a chromosome-scale genome assembly for the coral Acropora millepora. We then obtained whole genome sequences for 237 phenotyped samples collected at 12 reefs distributed along the Great Barrier Reef, among which we inferred very little population structure. Scanning the genome for evidence of local adaptation, we detected signatures of long-term balancing selection in the heat-shock co-chaperone sacsin. We further used 213 of the samples to conduct a genome-wide association study of visual bleaching score, in- corporating the polygenic score derived from it into a predictive model for bleaching in the wild. -
Porcine DNAJB6 Promotes PCV2 Replication Via Enhancing The
Han et al. Vet Res (2020) 51:61 https://doi.org/10.1186/s13567-020-00783-z RESEARCH ARTICLE Open Access Porcine DNAJB6 promotes PCV2 replication via enhancing the formation of autophagy in host cells Cong Han†, Qian Du†, Lei Zhu, Nannan Chen, Le Luo, Qiao Chen, Jiatong Yin, Xingchen Wu, Dewen Tong* and Yong Huang* Abstract Hsp40/DnaJ family proteins play important roles in the infection process of various viruses. Porcine DNAJB6 (pDNAJB6) is a major member of this family, but its role in modulating the replication of porcine circovirus type 2 (PCV2) is still unclear. In the present study, pDNAJB6 was found to be signifcantly upregulated by PCV2 infection, and confrmed to be interacted with PCV2 capsid (Cap) protein and co-localized at both cytoplasm and nucleus in the PCV2-infected cells. Knockout of pDNAJB6 signifcantly reduced the formation of autophagosomes in PCV2-infected cells or in the cells expressing Cap protein, whereas overexpression of pDNAJB6 showed an opposite efect. In addi- tion, the domain mapping assay showed that the J domain of pDNAJB6 (amino acids (aa) 1–99) and the C terminus of Cap (162-234 aa) were required for the interaction of pDNAJB6 with Cap. Notably, the interaction of pDNAJB6 with Cap was very important to promoting the formation of autophagosomes induced by PCV2 infection or Cap expression and enhancing the replication of PCV2. Taken together, the results presented here show a novel function of pDNAJB6 in regulation of porcine circovirus replication that pDNAJB6 enhances the formation of autophagy to promote viral replication through interacting with viral capsid protein during PCV2 infection. -
FOXP1 Acts Through a Negative Feedback Loop to Suppress FOXO-Induced Apoptosis
Cell Death and Differentiation (2013) 20, 1219–1229 & 2013 Macmillan Publishers Limited All rights reserved 1350-9047/13 www.nature.com/cdd FOXP1 acts through a negative feedback loop to suppress FOXO-induced apoptosis R van Boxtel1,5, C Gomez-Puerto1,6, M Mokry2,6, A Eijkelenboom3, KE van der Vos1, EES Nieuwenhuis2, BMT Burgering3, EW-F Lam4 and PJ Coffer*,1,2 Transcriptional activity of Forkhead box transcription factor class O (FOXO) proteins can result in a variety of cellular outcomes depending on cell type and activating stimulus. These transcription factors are negatively regulated by the phosphoinositol 3-kinase (PI3K)–protein kinase B (PKB) signaling pathway, which is thought to have a pivotal role in regulating survival of tumor cells in a variety of cancers. Recently, it has become clear that FOXO proteins can promote resistance to anti-cancer therapeutics, designed to inhibit PI3K–PKB activity, by inducing the expression of proteins that provide feedback at different levels of this pathway. We questioned whether such a feedback mechanism may also exist directly at the level of FOXO-induced transcription. To identify critical modulators of FOXO transcriptional output, we performed gene expression analyses after conditional activation of key components of the PI3K–PKB–FOXO signaling pathway and identified FOXP1 as a direct FOXO transcriptional target. Using chromatin immunoprecipitation followed by next-generation sequencing, we show that FOXP1 binds enhancers that are pre-occupied by FOXO3. By sequencing the transcriptomes of cells in which FOXO is specifically activated in the absence of FOXP1, we demonstrate that FOXP1 can modulate the expression of a specific subset of FOXO target genes, including inhibiting expression of the pro-apoptotic gene BIK. -
Weihl 234Th Workshop Report Weihl
Workshop Report 234th ENMC International Workshop: Chaperone dysfunction in muscle disease December 8-10th 2017, Naarden, Netherlands Conrad C. Weihl1, Bjarne Udd2, Michael Hanna3 on behalf of the Workshop Participants 1 Department of Neurology, Washington University School of Medicine, Saint Louis, MO USA 2 Tampere Neuromuscular Center and Folkhalsan Genetic Institute, Helsinki, Finland 3 UCL Institute of Neurology, Queen Square, London, UK 1. Introduction: Twenty participants from Australia, Belgium, Denmark, Finland, France, Germany, Italy, Israel, The Netherlands, Sweden, UK and the USA met in Naarden, The Netherlands from December 8th-10th, 2017. This group included clinicians, clinical trialists, basic scientists, industry and patient representatives. Patients were represented by the Muscular Dystrophy Association and Alexander’s Way (BAG3 associated myopathy). The goals were to discuss the role of protein chaperones in normal muscle function, muscle disease and future therapies. Protein chaperones are a class of proteins that participate in facilitating the proper folding and assembly of protein complexes. Thus, chaperones are essential for the development and maintenance of skeletal muscle. Specifically this large group of proteins ensures that other proteins (client proteins) maintain proper structure and function or if needed, facilitates their degradation via proteolytic pathways. Chaperone dysfunction is responsible for many rare hereditary myopathies; thus correcting chaperone function may be a therapeutic option. The participants reported on various aspects of the involvement of chaperones in a large variety of muscle diseases and disease processes, ranging from primary defects in chaperone genes such as DNAJB6, BAG3 and HSPB8, to the involvement of chaperones in the larger group of myofibrillar and rimmed vacuolar myopathies including sporadic inclusion body myositis (sIBM), and beyond. -
The HSP70 Chaperone Machinery: J Proteins As Drivers of Functional Specificity
REVIEWS The HSP70 chaperone machinery: J proteins as drivers of functional specificity Harm H. Kampinga* and Elizabeth A. Craig‡ Abstract | Heat shock 70 kDa proteins (HSP70s) are ubiquitous molecular chaperones that function in a myriad of biological processes, modulating polypeptide folding, degradation and translocation across membranes, and protein–protein interactions. This multitude of roles is not easily reconciled with the universality of the activity of HSP70s in ATP-dependent client protein-binding and release cycles. Much of the functional diversity of the HSP70s is driven by a diverse class of cofactors: J proteins. Often, multiple J proteins function with a single HSP70. Some target HSP70 activity to clients at precise locations in cells and others bind client proteins directly, thereby delivering specific clients to HSP70 and directly determining their fate. In their native cellular environment, polypeptides are participates in such diverse cellular functions. Their constantly at risk of attaining conformations that pre- functional diversity is remarkable considering that vent them from functioning properly and/or cause them within and across species, HSP70s have high sequence to aggregate into large, potentially cytotoxic complexes. identity. They share a single biochemical activity: an Molecular chaperones guide the conformation of proteins ATP-dependent client-binding and release cycle com- throughout their lifetime, preventing their aggregation bined with client protein recognition, which is typi- by protecting interactive surfaces against non-productive cally rather promiscuous. This apparent conundrum interactions. Through such inter actions, molecular chap- is resolved by the fact that HSP70s do not work alone, erones aid in the folding of nascent proteins as they are but rather as ‘HSP70 machines’, collaborating with synthesized by ribosomes, drive protein transport across and being regulated by several cofactors.