[Rh2(Esp)2]-Catalyzed Intermolecular C(Sp3)
Total Page:16
File Type:pdf, Size:1020Kb
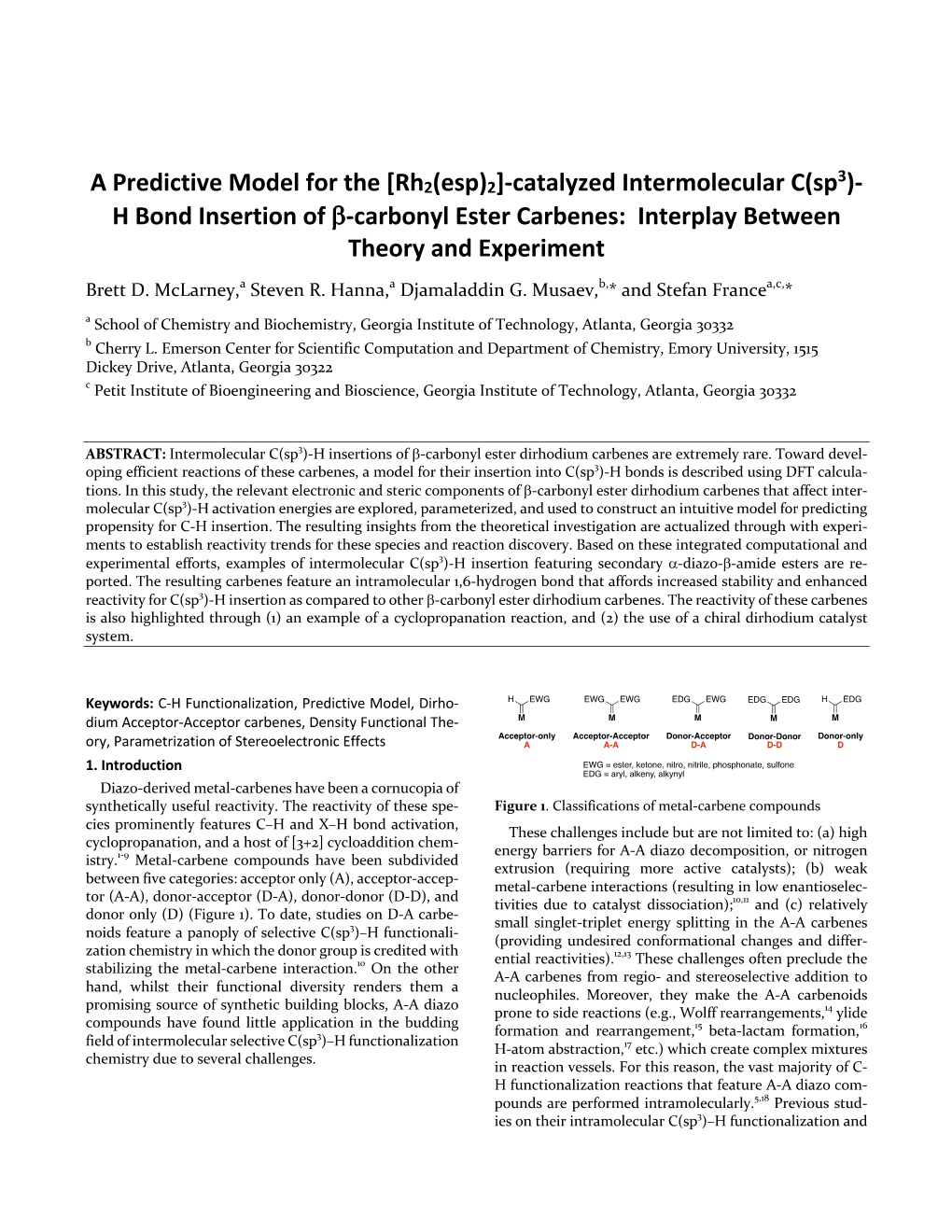
Load more
Recommended publications
-
Download The
The Final Program The Final Program for this Symposium was modified from that presented in the Book of Abstracts mainly due to the unforeseen incidence of the coronavirus in late January, 2020. We are grateful to the distinguished scientists who were able to take the place of those who were unable to come, and we regretted the absence of the distinguished scientists who had planned until the last hours to be with us. The SCHEDULE that is posted on this web site is the Final Schedule. That which is in the Book of Abstracts is the schedule that was expected to be the final schedule two weeks prior to the event. Strongly Donating 1,2,3-Triazole-Derived Carbenes for Metal-Mediated Redox Catalysis Simone Bertini, Matteo Planchestainer, Francesca Paradisi, Martin Albrecht Department of Chemistry & Biochemistry, University of Bern, CH-3012 Bern, Switzerland [email protected] Triazole-derived N-heterocyclic carbenes have become an attractive addition to the family of NHC ligands, in parts because their versatile and functional group-tolerant synthesis through click- chemistry,1 and in other parts because of their stronger donor properties to transition metals when compared to ubiquitous Arduengo-type carbenes.2. We have been particularly attracted recently by the high robustness of these ligands towards oxidative and reductive conditions, which provides appealing opportunities for challenging redox catalysis. We have exploited these properties for example for developing iridium complexes as highly active and molecular water oxidation catalysts.3 Here we will present our efforts to use triazole-derived carbenes to enable 1st row transition metals as catalytically competent entities. -
Certified by ...--- ,'
[4 + 1] ANNULATION REACTIONS OF (TRIALKYLSILYL)KETENES: SYNTHESIS OF SUBSTITUTED INDANONES AND CYCLOPENTENONES by Christopher P. Davie B.S., Chemistry Boston College, 1999 SUBMITTED TO THE DEPARTMENT OF CHEMISTRY IN PARTIAL FULFILLMENT OF THE REQUIREMENTS FOR THE DEGREE OF DOCTOR OF PHILOSOPHY at the MASSACHUSETTS INSTITUTE OF TECHNOLOGY September 2005 © 2005 Massachusetts Institute of Technology All Rights Reserved Signaure of Author....................... ......... epartmentof Chem..............................istry Department of Chemistry June 7, 2005 Certifiedby ........ ....--- ,'................................ ................. Rick L. Danheiser Thesis Supervisor Acceptedby.................................................................................................... Robert W. Field -- MASSACHUSETTS INSTIUTE Departmental committee on Uraduate Studies I OF TECHNOLOGY OCT 1 12005 ARCHIVEs. LIBRARIES This doctoral thesis has been examined by a committee of the Department of Chemistry as follows: Professor Gregory C. Fu I... ..............; Chairman ]ProfessorProfessor RickRick LL. Danheiser....~cDanheiser ...... ....... ............. .. .................................... Thesis Supervisor Professor Timothy F. Jamison ...... ..... 2 Acknowledgments I would first like to thank my advisor, Rick Danheiser, for his mentoring and support during the past five years. I greatly appreciate Rick's dedication to education, and all that I have learned from him and by working in his lab. I am grateful to Professors Greg Fu and Tim Jamison, members of my thesis committee, for their constructive feedback on this thesis as well as their assistance and advice during my job search. I must also thank Professor Fu for providing valuable advice during our annual meetings. I owe a debt of gratitude to the past and present members of the Danheiser group that I have had the pleasure of working with during my time at MIT. While chemistry was frustrating at times, days in lab were (almost) always enjoyable because of the great group of people working in the lab. -
Ketenes 25/01/2014 Part 1
Baran Group Meeting Hai Dao Ketenes 25/01/2014 Part 1. Introduction Ph Ph n H Pr3N C A brief history Cl C Ph + nPr NHCl Ph O 3 1828: Synthesis of urea = the starting point of modern organic chemistry. O 1901: Wedekind's proposal for the formation of ketene equivalent (confirmed by Staudinger 1911) Wedekind's proposal (1901) 1902: Wolff rearrangement, Wolff, L. Liebigs Ann. Chem. 1902, 325, 129. 2 Wolff adopt a ketene structure in 1912. R 2 hν R R2 1905: First synthesis and characterization of a ketene: in an efford to synthesize radical 2, 1 ROH R C Staudinger has synthesized diphenylketene 3, Staudinger, H. et al., Chem. Ber. 1905, 1735. N2 1 RO CH or Δ C R C R1 1907-8: synthesis and dicussion about structure of the parent ketene, Wilsmore, O O J. Am. Chem. Soc. 1907, 1938; Wilsmore and Stewart Chem. Ber. 1908, 1025; Staudinger and Wolff rearrangement (1902) O Klever Chem. Ber. 1908, 1516. Ph Ph Cl Zn Ph O hot Pt wire Zn Br Cl Cl CH CH2 Ph C C vs. C Br C Ph Ph HO O O O O O O O 1 3 (isolated) 2 Wilsmore's synthesis and proposal (1907-8) Staudinger's synthesis and proposal (1908) wanted to make Staudinger's discovery (1905) Latest books: ketene (Tidwell, 1995), ketene II (Tidwell, 2006), Science of Synthesis, Vol. 23 (2006); Latest review: new direactions in ketene chemistry: the land of opportunity (Tidwell et al., Eur. J. Org. Chem. 2012, 1081). Search for ketenes, Google gave 406,000 (vs. -
Carbene Rearrangements: Intramolecular Interaction of a Triple Bond with a Carbene Center
An Abstract OF THE THESIS OF Jose C. Danino for the degree of Doctor of Philosophy in Chemistry presented on _Dcc, Title: Carbene RearrangementE) Intramolecular Interaction of a Triple Bond with aCarbene Center Redacted for Privacy Abstract approved: Dr. Vetere. Freeman The tosylhydrazones of2-heptanone, 4,4-dimethy1-2- heptanone, 6-heptyn-2-one and 4,4-dimethy1-6-heptyn-2- one were synthesizedand decomposed under a varietyof reaction conditions:' drylithium and sodium salt pyrolyses, sodium methoxide thermolysesin diglyme and photolyses of the lithium salt intetrahydrofuran. The saturated ana- logues 2-heptanone tosylhydrazoneand its 4,4-dimethyl isomer afforded the alkenesarising from 6-hydrogeninser- product distribution in the tion. It was determined that differ- dry salt pyrolyses of2-heptanone tosylhydrazone was ent for the lithiumand the sodium salts. However, the product distribution of thedry sodium salt was verysimilar diglyme to product distributionobtained on thermolysis in explained by a with sodium methoxide. This difference was reaction of lithium bromide(present as an impurity inall compound to the lithium salts)with the intermediate diazo afford an organolithiumintermediate that behaves in a some- what different fashionthan the free carbene.The unsaturated analogues were found to produce a cyclic product in addition to the expected acyclic alkenes arising from 3-hydrogen insertion. By comparison of the acyclic alkene distri- bution obtained in the saturated analogues with those in the unsaturated analogues, it was concluded that at leastsome cyclization was occurring via addition of the diazo moiety to the triple bond. It was determined that the organo- lithium intermediateresulting from lithium bromide cat- alyzed decomposition of the diazo compound was incapable of cyclization. -
Reactions of (Trialkylsilyl)Vinylketenes with Lithium Ynolates: a New Benzannulation Strategy Wesley F. Austin, Yongjung Zhang
Reactions of (Trialkylsilyl)vinylketenes with Lithium Ynolates: A New Benzannulation Strategy Wesley F. Austin, Yongjung Zhang, and Rick L. Danheiser* Department of Chemistry Massachusetts Institute of Technology Cambridge, Massachusetts 02139 Supporting Information General Procedures. All reactions were performed in flame-dried or oven-dried glassware under a positive pressure of argon. Reaction mixtures were stirred magnetically unless otherwise indicated. Air- and moisture-sensitive liquids and solutions were transferred by syringe or cannula and were introduced into reaction vessels through rubber septa. Reaction product solutions and chromatography fractions were concentrated by rotary evaporation at ca. 20 mmHg and then at ca. 0.1 mmHg (vacuum pump) unless otherwise indicated. Thin layer chromatography was performed on Merck precoated glass- backed silica gel 60 F-254 0.25 mm plates. Column chromatography was performed on Silicycle silica gel 60 (230-400 mesh), or Sorbent silica gel 60A (32-63 µm). Materials. (Triisopropylsilyl)vinylketenes 6a and 6b were prepared as described previously.1 2- Cyclohexyl-1-(triisopropylsiloxy)ethyne (9a) and 3-methyl-1-(triisopropylsiloxy)-1-butyne (9b) were prepared from ethyl cyclohexanecarboxylate2 and ethyl isobutyrate3 respectively via the one-step Kowalski reaction as reported previously. Siloxy alkynes 9d4 and 9e5 have been prepared from the corresponding alkynes by employing the method of Julia.6 Anhydrous tert-butyl hydroperoxide in toluene was prepared from the aqueous solution as described by Sharpless.7 Commercial grade reagents and solvents were used without further purification except as indicated below. Triisopropylsilyl trifluoromethanesulfonate, 1,1,1,3,3,3-hexamethyldisilazane, hexanes, benzene, and 1 Loebach, J. L.; Bennett, D. M.; Danheiser, R. -
Studies Towards the Synthesis of the Core Structure of Sarains A, B, and C
T v v O T T V\ v r S - j si V V A ^ O Synthetic Studies Toward^he^Core Structure of Sarains A, B and C A Thesis Presented to the University of London in Partial Fulfilment of the Requirements for the Degree of Doctor of Philosophy Gurdeep Singh Nandra September 2004 Christopher Ingold Laboratories Department of Chemistry University College London London W ClH 0AJ SSSSSSSL ProQuest Number: 10014333 All rights reserved INFORMATION TO ALL USERS The quality of this reproduction is dependent upon the quality of the copy submitted. In the unlikely event that the author did not send a complete manuscript and there are missing pages, these will be noted. Also, if material had to be removed, a note will indicate the deletion. uest. ProQuest 10014333 Published by ProQuest LLC(2016). Copyright of the Dissertation is held by the Author. All rights reserved. This work is protected against unauthorized copying under Title 17, United States Code. Microform Edition © ProQuest LLC. ProQuest LLC 789 East Eisenhower Parkway P.O. Box 1346 Ann Arbor, Ml 48106-1346 Abstract Sarains A-C are marine alkaloids produced by the sponge Reniera sarai, which exhibit antitumor, antibacterial, and insecticidal activities. Sarains A-C have a complex structure, which comprises of a l,5-diazatetracyclo[6.2.1.1^’^.0'^’*^]dodecane tricyclic core surrounded by two macrocycles. To date four groups have communicated the construction of the tricyclic core, however the total synthesis of sarains A-C has yet to be reported. This thesis describes the work undertaken towards the synthesis of a tricycle that contains the core structure of sarains A-C, by the use of a novel carbene mediated ylide rearrangement reaction. -
Recent Advances in Enantioselective Photochemical Reactions of Stabilized Diazo Compounds
molecules Review Recent Advances in Enantioselective Photochemical Reactions of Stabilized Diazo Compounds Ting-Bi Hua, Qing-Qing Yang * and You-Quan Zou y College of Materials and Chemical Engineering, Key Laboratory of Inorganic Nonmetallic Crystalline and Energy Conversion Materials, China Three Gorges University, 8 Daxue Road, Yichang 443002, Hubei, China * Correspondence: [email protected] Current address: Department of Organic Chemistry, Weizmann Institute of Science, Rehovot 76100, Israel. y Received: 3 August 2019; Accepted: 26 August 2019; Published: 2 September 2019 Abstract: Diazo compounds have proven to be a useful class of carbenes or metal carbenoids sources under thermal, photochemical, or metal-catalyzed conditions, which can subsequently undergo a wide range of synthetically important transformations. Recently, asymmetric photocatalysis has provoked increasing research interests, and great advances have been made in this discipline towards the synthesis of optically enriched compounds. In this context, the past two decades have been the most productive period in the developments of enantioselective photochemical reactions of diazo compounds due to a better understanding of the reactivities of diazo compounds and the emergence of new catalytic modes, as well as easier access to and treatment of stabilized diazo compounds. This review highlights these impressive achievements according to the reaction type, and the general mechanisms and stereochemical inductions are briefly discussed as well. Keywords: diazo compounds; enantioselectivity; photocatalysis; Wolff rearrangement 1. Introduction Diazo compounds are a class of charge neutral organic compounds containing a diazo group bound to a carbon atom, which can resonance into different structures (Figure1a) [ 1]. Since its chemistry has existed for more than a century [2], diazo compounds have been rendered to be versatile building blocks and will continue to play an important role in organic synthesis. -
© Cambridge University Press Cambridge
Cambridge University Press 0521770971 - Modern Methods of Organic Synthesis W. Carruthers and Iain Coldham Index More information Index acidity 1 from alkynes 125–32 acyl anion equivalents 56 from diols 123 acyloin reaction 425 from hydrazones 120 Adams’ catalyst 407 reaction of AD-mix ␣ 352 with carbenes 303–9 AD-mix  352 with dienes in Diels–Alder reaction 162 agelastatin A 376 with radicals 280–98 AIBN 268 reduction of 322, 408–13, 459 alane 437, 444 alkenyllithium species 57, 59 alcohols alkylation 1–19 deoxygenation 270 asymmetric 37 from alkenes 323, 349 with enamines 1, 17 from carbonyl compounds 416, 421, 423, 434–56 with enolates 1–16 oxidation 378–93 with metalloenamines 16 aldehydes alkyl halides alkylation of 17 oxidation to carbonyl compounds 384 as dienophiles in Diels–Alder reaction 169 reductive cleavage to hydrocarbons 269, 406, 442 decarbonylation of 419 alkyllithium species 46 from alcohols 380 alkynes from alkenes 325, 360, 364 conversion to alkenes 125–32, 414 oxidation of 392 deprotonation of 58 reduction of 435, 439, 443 hydrometallation 128 reductive dimerization of 148, 425 preparation of 137 Alder–ene reaction 231 reduction of 125 aldol reaction 27–36 allopumiliotoxin 58 diastereoselective 32 allosamidin disaccharides 272 enantioselective 41 allylic organometallics 71–4 aldosterone 276 allylic oxidation 374 alkenes allylic 1,3-strain 26, 73, 351 allylic oxidation of 374 -allylpalladium complexes 98 conversion to alcohols 323 amabiline 220 conversion to ketones (Wacker reaction) 365 ambruticin S 308 epoxidation -
Molecular REARRANGEMENTS
Key words: rearrangement reactions, migration to electron deficient nitrogen, electron deficient oxygen, electron deficient carbon. Migratory aptitude, cross- over experiments Rearrangment reactions are an interesting class of reactions wherein a group or an atom migration during the course of the reaction. While most of the rearrangements are designed in that fashion, it can also be undesirable in some cases. Depending on the reaction conditions, the nature of rearrangement (and the product) could also change. In this module, various rearrangement reactions are presented. These are classified with respect the the migration origin and migration terminus. Emphasis has been placed on examples involving skeletal rearrangements that are practically used in day-to-day organic synthesis. Rearrangement reactions involve the migration of a group or an atom from one center (migration origin) to another (migration terminus) within the same molecule. W W A B A B In the above-mentioned generalized representation, atom-A is migration origin from where the migrating group “W” moves to atom-B (migration terminus) These rearrangements can be roughly classified on the basis of the nature of the migrating group/atom, i.Nucleophilic or Anionotropic: migrating group migrates with its electron pair. ii.Electrophilic or cationotropic: migrating group migrates without its electron pair. iii.Free radical: migrating group migrates with only one electron. Of these most commonly found are nucleophilic one. These rearrangements can take place in two possible modes, i.Intramolecular : In these migrating group do not completely detach from the migration origin and occurs within the same molecule. W A B A B W ii. Intermolecular : In these migrating group is detached from the migration origin. -
(II) Catalyzed Wolff Rearrangement of a Carbenoid Derived from an Indolyl Diazopropanedione
Rev. Soc. Quím. Méx. 2004, 48, 46-48 Investigación Rhodium (II) Catalyzed Wolff Rearrangement of a Carbenoid Derived from an Indolyl Diazopropanedione Erick Cuevas Yañez* and Raymundo Cruz Almanza† Instituto de Química de la Universidad Nacional Autónoma de México. Circuito Exterior, Ciudad Universitaria, Coyoacán, 04510, México, D.F. Tel. (52) 56 22 44 08; fax (52) 56 16 22 17. *email: [email protected] †Deceased, October 2003. Recibido 27 de febrero del 2004; aceptado el 31 de marzo del 2004. Abstract. The Rhodium (II) acetate catalyzed reaction of 3-diazo-1- Resumen. La reacción catalizada por acetato de rodio (II) de la 3- (indol-3-yl)- propane-1,2-dione did not give the expected intramolec- diazo-1-(3-indoil)-1,2-propanodiona no dio el producto de ciclación ular cyclization product, affording only 3-acetylindole in 70% yield. intramolecular esperado, generando solamente 3-acetilindol en un A reaction mechanism which involves a Wolff rearrangement is pro- rendimiento del 70% . Se propone un mecanismo de reacción que posed. involucra una transposición de Wolff. Key Words: Carbenoid, rearrangement, indole. Palabras Clave: Carbenoide, transposición, indol. Introduction instead of the carbenoid insertion. Therefore, this is the first report on a Wolff type rearrangement in carbenoids derived The diazo group chemistry has taken a new interest as a conse- from 3-diazoalkanoylindoles. quence of the development of catalytic methods with transi- tion metals which particularly convert diazoketones in to valu- able tools in organic synthesis with several applications in Results and discussion homologations, X-H bond insertions, ylide formations, and reactions with alkenes and aromatic compounds [1]. -
Cyclic Enaminones: Methodology Development, Total Synthesis, and Library Construction a Dissertation Submitted to the Faculty Of
CYCLIC ENAMINONES: METHODOLOGY DEVELOPMENT, TOTAL SYNTHESIS, AND LIBRARY CONSTRUCTION A DISSERTATION SUBMITTED TO THE FACULTY OF THE GRADUATE SCHOOL OF THE UNIVERSITY OF MINNESOTA BY HAJIME SEKI IN PARTIAL FULFILLMENT OF THE REQUIREMENTS FOR THE DEGREE OF DOCTOR OF PHILOSOPHY GUNDA I. GEORG DECEMBER 2011 ©2011 Hajime Seki ACKNOWLEDGEMENTS Looking back my life as a graduate student, it was undoubtedly one of my best decisions in my life to study chemistry in the University of Minnesota. The path I chose to come to the U.S. from Japan, leaving behind all of my family, friends, and comfortable environments, was certainly not the easiest trail that one could go through. During the course of my graduate study, however, a countless people supported me, and taught me things that I would not be able to learn in Japan, such as an appreciation to the diversity and how to be an independent individual/scientist. It was also the first opportunity to see Japan from outside and realize where it stands in the world. All the things I have learned will definitely be my invaluable treasures in the future. Herein, I would like to acknowledge the people who have stood by me. I owe my first acknowledgement to my parents for their unconditional love. It was their encouragements and dedications that I was able to step out of Japan and study in the U.S. I thank my academic advisor, Prof. Gunda I. Georg, for her guidance and support. On a snowy day of Jan. 15th in 2007, she kindly welcomed me in her group. -
Addition of Silylated Nucleophiles to Α-Oxoketenes Yohan Dudognon, Marc Presset, Jean Rodriguez, Yoann Coquerel, Xavier Bugaut, Thierry Constantieux
Addition of silylated nucleophiles to α-oxoketenes Yohan Dudognon, Marc Presset, Jean Rodriguez, Yoann Coquerel, Xavier Bugaut, Thierry Constantieux To cite this version: Yohan Dudognon, Marc Presset, Jean Rodriguez, Yoann Coquerel, Xavier Bugaut, et al.. Addition of silylated nucleophiles to α-oxoketenes. Chemical Communications, Royal Society of Chemistry, 2016, 52 (14), pp.3010-3013. 10.1039/C5CC10217K. hal-01323402 HAL Id: hal-01323402 https://hal.archives-ouvertes.fr/hal-01323402 Submitted on 28 Mar 2017 HAL is a multi-disciplinary open access L’archive ouverte pluridisciplinaire HAL, est archive for the deposit and dissemination of sci- destinée au dépôt et à la diffusion de documents entific research documents, whether they are pub- scientifiques de niveau recherche, publiés ou non, lished or not. The documents may come from émanant des établissements d’enseignement et de teaching and research institutions in France or recherche français ou étrangers, des laboratoires abroad, or from public or private research centers. publics ou privés. Distributed under a Creative Commons Attribution - NonCommercial| 4.0 International License ChemComm View Article Online COMMUNICATION View Journal | View Issue Addition of silylated nucleophiles to a-oxoketenes† Cite this: Chem. Commun., 2016, 52,3010 Yohan Dudognon, Marc Presset,‡ Jean Rodriguez, Yoann Coquerel,* Received 11th December 2015, Xavier Bugaut* and Thierry Constantieux* Accepted 13th January 2016 DOI: 10.1039/c5cc10217k www.rsc.org/chemcomm A general evaluation of silylated nucleophiles to intercept transient those nucleophiles are gases, often with high toxicities; (iii) a-oxoketenes generated by microwave-assisted Wolff rearrange- product stability since the resulting naked functionalities in the ment of 2-diazo-1,3-dicarbonyl compounds is presented.