The Outer Heliosphere – Solar System's Final Frontier V
Total Page:16
File Type:pdf, Size:1020Kb
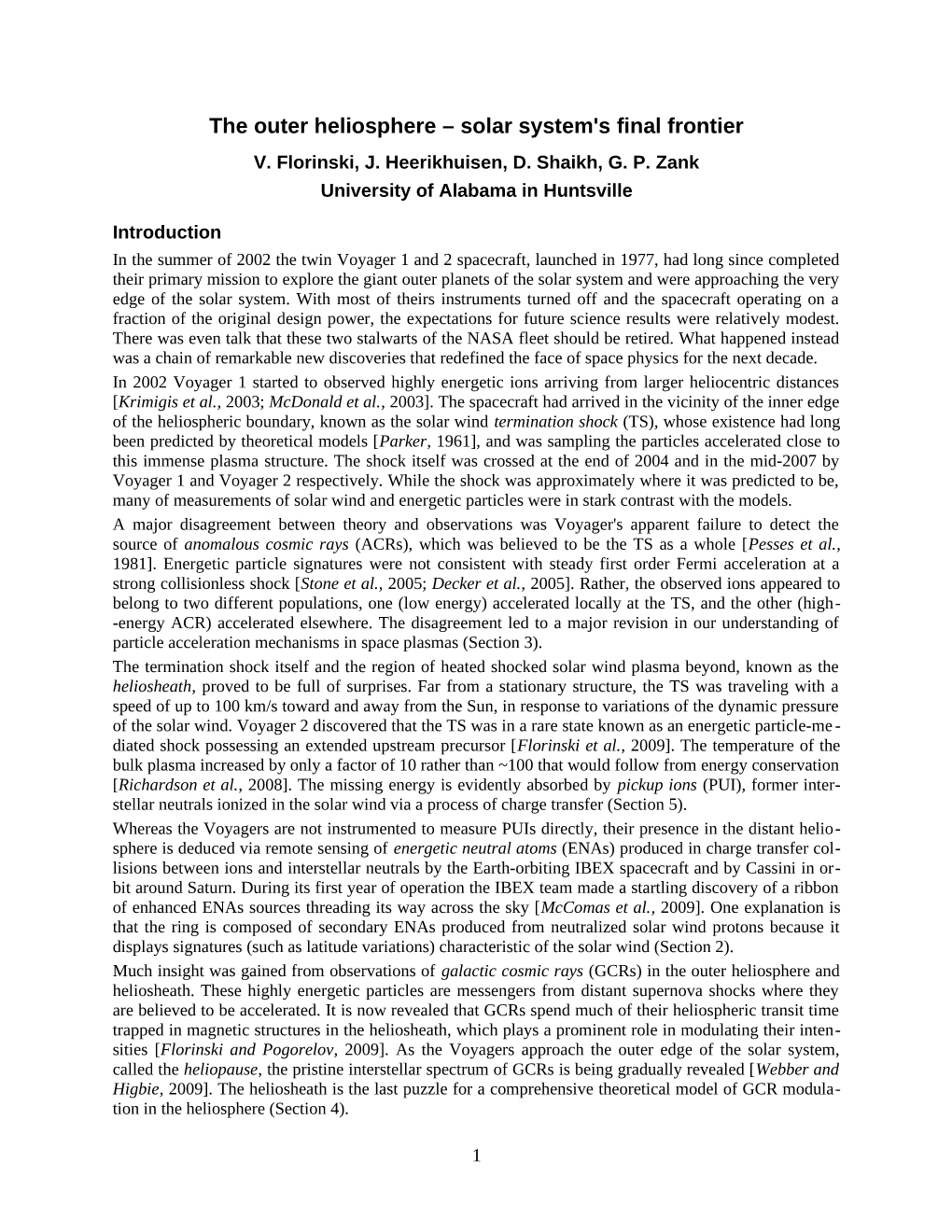
Load more
Recommended publications
-
Introduction to Astronomy from Darkness to Blazing Glory
Introduction to Astronomy From Darkness to Blazing Glory Published by JAS Educational Publications Copyright Pending 2010 JAS Educational Publications All rights reserved. Including the right of reproduction in whole or in part in any form. Second Edition Author: Jeffrey Wright Scott Photographs and Diagrams: Credit NASA, Jet Propulsion Laboratory, USGS, NOAA, Aames Research Center JAS Educational Publications 2601 Oakdale Road, H2 P.O. Box 197 Modesto California 95355 1-888-586-6252 Website: http://.Introastro.com Printing by Minuteman Press, Berkley, California ISBN 978-0-9827200-0-4 1 Introduction to Astronomy From Darkness to Blazing Glory The moon Titan is in the forefront with the moon Tethys behind it. These are two of many of Saturn’s moons Credit: Cassini Imaging Team, ISS, JPL, ESA, NASA 2 Introduction to Astronomy Contents in Brief Chapter 1: Astronomy Basics: Pages 1 – 6 Workbook Pages 1 - 2 Chapter 2: Time: Pages 7 - 10 Workbook Pages 3 - 4 Chapter 3: Solar System Overview: Pages 11 - 14 Workbook Pages 5 - 8 Chapter 4: Our Sun: Pages 15 - 20 Workbook Pages 9 - 16 Chapter 5: The Terrestrial Planets: Page 21 - 39 Workbook Pages 17 - 36 Mercury: Pages 22 - 23 Venus: Pages 24 - 25 Earth: Pages 25 - 34 Mars: Pages 34 - 39 Chapter 6: Outer, Dwarf and Exoplanets Pages: 41-54 Workbook Pages 37 - 48 Jupiter: Pages 41 - 42 Saturn: Pages 42 - 44 Uranus: Pages 44 - 45 Neptune: Pages 45 - 46 Dwarf Planets, Plutoids and Exoplanets: Pages 47 -54 3 Chapter 7: The Moons: Pages: 55 - 66 Workbook Pages 49 - 56 Chapter 8: Rocks and Ice: -
A Deep Learning Framework for Solar Phenomena Prediction
FlareNet: A Deep Learning Framework for Solar Phenomena Prediction FDL 2017 Solar Storm Team: Sean McGregor1, Dattaraj Dhuri1,2, Anamaria Berea1,3, and Andrés Muñoz-Jaramillo1,4 1NASA’s 2017 Frontier Development Laboratory 2Tata Institute of Fundamental Research (TIFR), Mumbai, India 3Center for Complexity in Business, University of Maryland, College Park, MD, USA 4SouthWest Research Institute, Boulder, CO, USA Abstract Solar activity can interfere with the normal operation of GPS satellites, the power grid, and space operations, but inadequate predictive models mean we have little warning for the arrival of newly disruptive solar activity. Petabytes of data col- lected from satellite instruments aboard the Solar Dynamics Observatory (SDO) provide a high-cadence, high-resolution, and many-channeled dataset of solar phenomena. Several challenging deep learning problems may be derived from the data, including space weather forecasting (i.e., solar flares, solar energetic particles, and coronal mass ejections). This work introduces a software framework, FlareNet, for experimentation within these problems. FlareNet includes compo- nents for the downloading and management of SDO data, visualization, and rapid experimentation. The system architecture is built to enable collaboration between heliophysicists and machine learning researchers on the topics of image regres- sion, image classification, and image segmentation. We specifically highlight the problem of solar flare prediction and offer insights from preliminary experiments. 1 Introduction The violent release of solar magnetic energy – collectively referred to as “space weather" – is responsible for a variety of phenomena that can disrupt technological assets. In particular, solar flares (sudden brightenings of the solar corona) and coronal mass ejections (CMEs; the violent release of solar plasma) can disrupt long-distance communications, reduce Global Positioning System (GPS) accuracy, degrade satellites, and disrupt the power grid [5]. -
Solar and Space Physics: a Science for a Technological Society
Solar and Space Physics: A Science for a Technological Society The 2013-2022 Decadal Survey in Solar and Space Physics Space Studies Board ∙ Division on Engineering & Physical Sciences ∙ August 2012 From the interior of the Sun, to the upper atmosphere and near-space environment of Earth, and outwards to a region far beyond Pluto where the Sun’s influence wanes, advances during the past decade in space physics and solar physics have yielded spectacular insights into the phenomena that affect our home in space. This report, the final product of a study requested by NASA and the National Science Foundation, presents a prioritized program of basic and applied research for 2013-2022 that will advance scientific understanding of the Sun, Sun- Earth connections and the origins of “space weather,” and the Sun’s interactions with other bodies in the solar system. The report includes recommendations directed for action by the study sponsors and by other federal agencies—especially NOAA, which is responsible for the day-to-day (“operational”) forecast of space weather. Recent Progress: Significant Advances significant progress in understanding the origin from the Past Decade and evolution of the solar wind; striking advances The disciplines of solar and space physics have made in understanding of both explosive solar flares remarkable advances over the last decade—many and the coronal mass ejections that drive space of which have come from the implementation weather; new imaging methods that permit direct of the program recommended in 2003 Solar observations of the space weather-driven changes and Space Physics Decadal Survey. For example, in the particles and magnetic fields surrounding enabled by advances in scientific understanding Earth; new understanding of the ways that space as well as fruitful interagency partnerships, the storms are fueled by oxygen originating from capabilities of models that predict space weather Earth’s own atmosphere; and the surprising impacts on Earth have made rapid gains over discovery that conditions in near-Earth space the past decade. -
From the Heliosphere Into the Sun Programme Book Incuding All
511th WE-Heraeus-Seminar From the Heliosphere into the Sun –SailingagainsttheWind– Programme book incuding all abstracts Physikzentrum Bad Honnef, Germany January 31 – February 3, 2012 http://www.mps.mpg.de/meetings/heliocorona/ From the Heliosphere into the Sun A meeting dedicated to the progress of our understanding of the solar wind and the corona in the light of the upcoming Solar Orbiter mission This meeting is dedicated to the processes in the solar wind and corona in the light of the upcoming Solar Orbiter mission. Over the last three decades there has been astonishing progress in our understanding of the solar corona and the inner heliosphere driven by remote-sensing and in-situ observations. This period of time has seen the first high-resolution X-ray and EUV observations of the corona and the first detailed measurements of the ion and electron velocity distribution functions in the inner heliosphere. Today we know that we have to treat the corona and the wind as one single object, which calls for a mission that is fully designed to investigate the interwoven processes all the way from the solar surface to the heliosphere. The meeting will provide a forum to review the advances over the last decades, relate them to our current understanding and to discuss future directions. We will concentrate one day on in- situ observations and related models of the inner heliosphere, and spend another day on remote sensing observations and modeling of the corona – always with an eye on the symbiotic nature of the two. On the third day we will direct our view towards the future. -
Exploring the Sun with ALMA
Astronomical Science DOI: 10.18727/0722-6691/5065 Exploring the Sun with ALMA Timothy S. Bastian1 18 Space Vehicles Directorate, Air Force and to gain an understanding of how Miroslav Bárta2 Research Laboratory, Albuquerque, mechanical and radiative energy are Roman Brajša3 USA transferred through that atmospheric Bin Chen4 19 National Astronomical Observatories, layer. Bart De Pontieu5, 6 Chinese Academy of Sciences, Beijing, Dale E. Gary4 China Much of what is currently known about Gregory D. Fleishman4 the chromosphere has relied on spectro- Antonio S. Hales1, 7 scopic observations at optical and ultra- Kazumasa Iwai8 The Atacama Large Millimeter/submilli- violet wavelengths using both ground- Hugh Hudson9, 10 meter Array (ALMA) Observatory opens and space-based instrumentation. While Sujin Kim11, 12 a new window onto the Universe. The a lot of progress has been made, the Adam Kobelski13 ability to perform continuum imaging interpretation of such observations is Maria Loukitcheva4, 14, 15 and spectroscopy of astrophysical phe- complex because optical and ultraviolet Masumi Shimojo16, 17 nomena at millimetre and submillimetre lines in the chromosphere form under Ivica Skokić2, 3 wavelengths with unprecedented sen- conditions of non-local thermodynamic Sven Wedemeyer6 sitivity opens up new avenues for the equilibrium. In contrast, emission from Stephen M. White18 study of cosmology and the evolution the Sun’s chromosphere at millimetre Yihua Yan19 of galaxies, the formation of stars and and submillimetre wavelengths is more planets, and astrochemistry. ALMA also straightforward to interpret as the emis- allows fundamentally new observations sion forms under conditions of local 1 National Radio Astronomy Observatory, to be made of objects much closer to thermodynamic equilibrium and the Charlottesville, USA home, including the Sun. -
Science Objectives May Be Summarized As Follows
MAGNETOSPHERE IMAGING INSTRUMENT (MIMI) 9 ON THE CASSINI MISSION TO SATURN/TITAN 2. Scientific Objectives MIMI science objectives may be summarized as follows: Saturn • Determine the global configuration and dynamics of hot plasma in the magneto- sphere of Saturn through energetic neutral particle imaging of ring current, radia- tion belts, and neutral clouds. • Study the sources of plasmas and energetic ions through in situ measurements of energetic ion composition, spectra, charge state, and angular distributions. • Search for, monitor, and analyze magnetospheric substorm-like activity at Saturn. • Determine through the imaging and composition studies the magnetosphere– satellite interactions at Saturn and understand the formation of clouds of neutral hydrogen, nitrogen, and water products. •Investigate the modification of satellite surfaces and atmospheres through plasma and radiation bombardment. • Study Titan’s cometary interaction with Saturn’s magnetosphere (and the solar wind) via high-resolution imaging and in situ ion and electron measurements. • Measure the high energy (Ee > 1 MeV, Ep > 15 MeV) particle component in the inner (L < 5 RS) magnetosphere to assess cosmic ray albedo neutron decay (CRAND) source characteristics. •Investigate the absorption of energetic ions and electrons by the satellites and rings in order to determine particle losses and diffusion processes within the mag- netosphere. • Study magnetosphere–ionosphere coupling through remote sensing of aurora and in situ measurements of precipitating energetic ions and electrons. Jupiter • Study ring current(s), plasma sheet, and neutral clouds in the magnetosphere and magnetotail of Jupiter during Cassini flyby, using global imaging and in situ mea- surements. S. M. KRIMIGIS ET AL. 10 Interplanetary • Determine elemental and isotopic composition of local interstellar medium through measurements of interstellar pickup ions. -
Chapter 11 RADIO OBSERVATIONS of CORONAL MASS
Chapter 11 RADIO OBSERVATIONS OF CORONAL MASS EJECTIONS Angelos Vourlidas Solar Physics Branch, Naval Research Laboratory, Washington, DC [email protected] Abstract In this chapter we review the status of CME observations in radio wavelengths with an emphasis on imaging. It is an area of renewed interest since 1996 due to the upgrade of the Nancay radioheliograph in conjunction with the continu- ous coverage of the solar corona from the EIT and LASCO instruments aboard SOHO. Also covered are analyses of Nobeyama Radioheliograph data and spec- tral data from a plethora of spectrographs around the world. We will point out the shortcomings of the current instrumentation and the ways that FASR could contribute. A summary of the current understanding of the physical processes that are involved in the radio emission from CMEs will be given. Keywords: Sun: Corona, Sun: Coronal Mass Ejections, Sun:Radio 1. Coronal Mass Ejections 1.1 A Brief CME Primer A Coronal Mass Ejection (CME) is, by definition, the expulsion of coronal plasma and magnetic field entrained therein into the heliosphere. The event is detected in white light by Thompson scattering of the photospheric light by the coronal electrons in the ejected mass. The first CME was discovered on December 14, 1971 by the OSO-7 orbiting coronagraph (Tousey 1973) which recorded only a small number of events (Howard et al. 1975). Skylab ob- servations quickly followed and allowed the first study of CME properties ( Gosling et al. 1974). Observations from many thousands of events have been collected since, from a series of space-borne coronagraphs: Solwind (Michels et al. -
Solar Wind Properties and Geospace Impact of Coronal Mass Ejection-Driven Sheath Regions: Variation and Driver Dependence E
Solar Wind Properties and Geospace Impact of Coronal Mass Ejection-Driven Sheath Regions: Variation and Driver Dependence E. K. J. Kilpua, D. Fontaine, C. Moissard, M. Ala-lahti, E. Palmerio, E. Yordanova, S. Good, M. M. H. Kalliokoski, E. Lumme, A. Osmane, et al. To cite this version: E. K. J. Kilpua, D. Fontaine, C. Moissard, M. Ala-lahti, E. Palmerio, et al.. Solar Wind Properties and Geospace Impact of Coronal Mass Ejection-Driven Sheath Regions: Variation and Driver Dependence. Space Weather: The International Journal of Research and Applications, American Geophysical Union (AGU), 2019, 17 (8), pp.1257-1280. 10.1029/2019SW002217. hal-03087107 HAL Id: hal-03087107 https://hal.archives-ouvertes.fr/hal-03087107 Submitted on 23 Dec 2020 HAL is a multi-disciplinary open access L’archive ouverte pluridisciplinaire HAL, est archive for the deposit and dissemination of sci- destinée au dépôt et à la diffusion de documents entific research documents, whether they are pub- scientifiques de niveau recherche, publiés ou non, lished or not. The documents may come from émanant des établissements d’enseignement et de teaching and research institutions in France or recherche français ou étrangers, des laboratoires abroad, or from public or private research centers. publics ou privés. RESEARCH ARTICLE Solar Wind Properties and Geospace Impact of Coronal 10.1029/2019SW002217 Mass Ejection-Driven Sheath Regions: Variation and Key Points: Driver Dependence • Variation of interplanetary properties and geoeffectiveness of CME-driven sheaths and their dependence on the E. K. J. Kilpua1 , D. Fontaine2 , C. Moissard2 , M. Ala-Lahti1 , E. Palmerio1 , ejecta properties are determined E. -
Planetary Magnetospheres
CLBE001-ESS2E November 9, 2006 17:4 100-C 25-C 50-C 75-C C+M 50-C+M C+Y 50-C+Y M+Y 50-M+Y 100-M 25-M 50-M 75-M 100-Y 25-Y 50-Y 75-Y 100-K 25-K 25-19-19 50-K 50-40-40 75-K 75-64-64 Planetary Magnetospheres Margaret Galland Kivelson University of California Los Angeles, California Fran Bagenal University of Colorado, Boulder Boulder, Colorado CHAPTER 28 1. What is a Magnetosphere? 5. Dynamics 2. Types of Magnetospheres 6. Interaction with Moons 3. Planetary Magnetic Fields 7. Conclusions 4. Magnetospheric Plasmas 1. What is a Magnetosphere? planet’s magnetic field. Moreover, unmagnetized planets in the flowing solar wind carve out cavities whose properties The term magnetosphere was coined by T. Gold in 1959 are sufficiently similar to those of true magnetospheres to al- to describe the region above the ionosphere in which the low us to include them in this discussion. Moons embedded magnetic field of the Earth controls the motions of charged in the flowing plasma of a planetary magnetosphere create particles. The magnetic field traps low-energy plasma and interaction regions resembling those that surround unmag- forms the Van Allen belts, torus-shaped regions in which netized planets. If a moon is sufficiently strongly magne- high-energy ions and electrons (tens of keV and higher) tized, it may carve out a true magnetosphere completely drift around the Earth. The control of charged particles by contained within the magnetosphere of the planet. -
Lectures 6, 7 and 8 October 8, 10 and 13 the Heliosphere
ESSESS 77 LecturesLectures 6,6, 77 andand 88 OctoberOctober 8,8, 1010 andand 1313 TheThe HeliosphereHeliosphere The Exploding Sun • We have seen that at times the Sun explosively sends plasma into the surrounding space. • This occurs most dramatically during CMEs. The History of the Solar Wind • 1878 Becquerel (won Noble prize for his discovery of radioactivity) suggests particles from the Sun were responsible for aurora • 1892 Fitzgerald (famous Irish Mathematician) suggests corpuscular radiation (from flares) is responsible for magnetic storms The Sun’s Atmosphere Extends far into Space 2008 Image 1919 Negative The Sun’s Atmosphere Extends Far into Space • The image of the solar corona in the last slide was taken with a natural occulting disk – the moon’s shadow. • The moon’s shadow subtends the surface of the Sun. • That the Sun had a atmosphere that extends far into space has been know for centuries- we are actually seeing sunlight scattered off of electrons. A Solar Wind not a Stationary Atmosphere • The Earth’s atmosphere is stationary. The Sun’s atmosphere is not stable but is blown out into space as the solar wind filling the solar system and then some. • The first direct measurements of the solar wind were in the 1960’s but it had already been suggested in the early 1900s. – To explain a correlation between auroras and sunspots Birkeland [1908] suggested continuous particle emission from these spots. – Others suggested that particles were emitted from the Sun only during flares and that otherwise space was empty [Chapman and Ferraro, 1931]. Discovery of the Solar Wind • That it is continuously expelled as a wind (the solar wind) was realized when Biermann [1951] noticed that comet tails pointed away from the Sun even when the comet was moving away from the Sun. -
Alabama Research Experiences for Undergraduates (ALREU)
Alabama Research Experiences for Undergraduates (ALREU) Project Title: Space Plasma Physics: Pickup ions, turbulence, solar wind, and waves Project Reference Code: UAH-Zank Hosting Institution: The University of Alabama in Huntsville Hosting Institution Location: Huntsville, AL Project Description: The Sun plays a major role in space plasma physics. The atmosphere of the Sun expands outwards at a supersonic velocity, which we call the solar wind, until the ram pressure of the solar wind is balanced by the interstellar medium pressure. When the expansion of the solar wind stops, a bubble-like region of space is formed in the interstellar medium, known as the heliosphere. The solar wind provides a unique opportunity to study a variety of plasma processes. In the solar wind, waves and turbulence occur everywhere, and is advected by the solar wind flow. Similarly, we can also observe unusual charged particles called pickup ions (PUIs) in the solar wind. PUIs are created by charge exchange between solar wind protons and interstellar neutral atoms that drift from the interstellar medium into the heliosphere. Waves, turbulence, and PUIs have particular properties and can modify the physics of the heliosphere, and the heliospheric termination shock (HTS). Here, we divide our project into four subprojects. The projects are related to investigating the pickup ion distribution function in the distant solar wind, turbulence in the solar wind, solar wind simulations, and waves in the solar wind. The student is free to choose any one of the projects. Students will be involved with state-of-the-art research under the general direction of Dr. -
A BRIEF HISTORY of CME SCIENCE 1. Introduction the Key to Understanding Solar Activity Lies in the Sun's Ever-Changing Magnetic
A BRIEF HISTORY OF CME SCIENCE 1 2 DAVID ALEXANDER , IAN G. RICHARDSON and THOMAS H. ZURBUCHEN3 1Department of Physics and Astronomy, Rice University, 6100 Main St., Houston, TX 77005, USA 2 The Astroparticle Physics Laboratory, NASA GSFC, Greenbelt, MD 20771, USA 3 Department of AOSS, University of Michigan, Ann Arbor, M148109, USA Received: 15 July 2004; Accepted in final form: 5 May 2005 Abstract. We present here a brief summary of the rich heritage of observational and theoretical research leading to the development of our current understanding of the initiation, structure, and evolution of Coronal Mass Ejections. 1. Introduction The key to understanding solar activity lies in the Sun's ever-changing magnetic field. The potential role played by the magnetic field in the solar atmosphere was first suggested by Frank Bigelow in 1889 after noting that the structure of the solar minimum corona seen during the eclipse of 1878 displayed marked equatorial extensions, called 'streamers '. Bigelow(1 890) noted th at the coronal streamers had a strong resemblance to magnetic lines of force and proposed th at the Sun must, in fact, be a large magnet. Subsequently, Hen ri Deslandres (1893) suggested that the forms and motions of prominences seen during so lar eclipses appeared to be influenced by a solar magnetic field. The link between magnetic fields and plasma emitted by the Sun was beginning to take shape by the turn of the 20th Century. The epochal discovery of magnetic fields on the Sun by American astronomer George Ellery Hale (1908) signalled the birth of modem solar physics.