Spatiotemporal Variation of Van Der Burgh's Coefficient in a Salt Plug Estuary
Total Page:16
File Type:pdf, Size:1020Kb
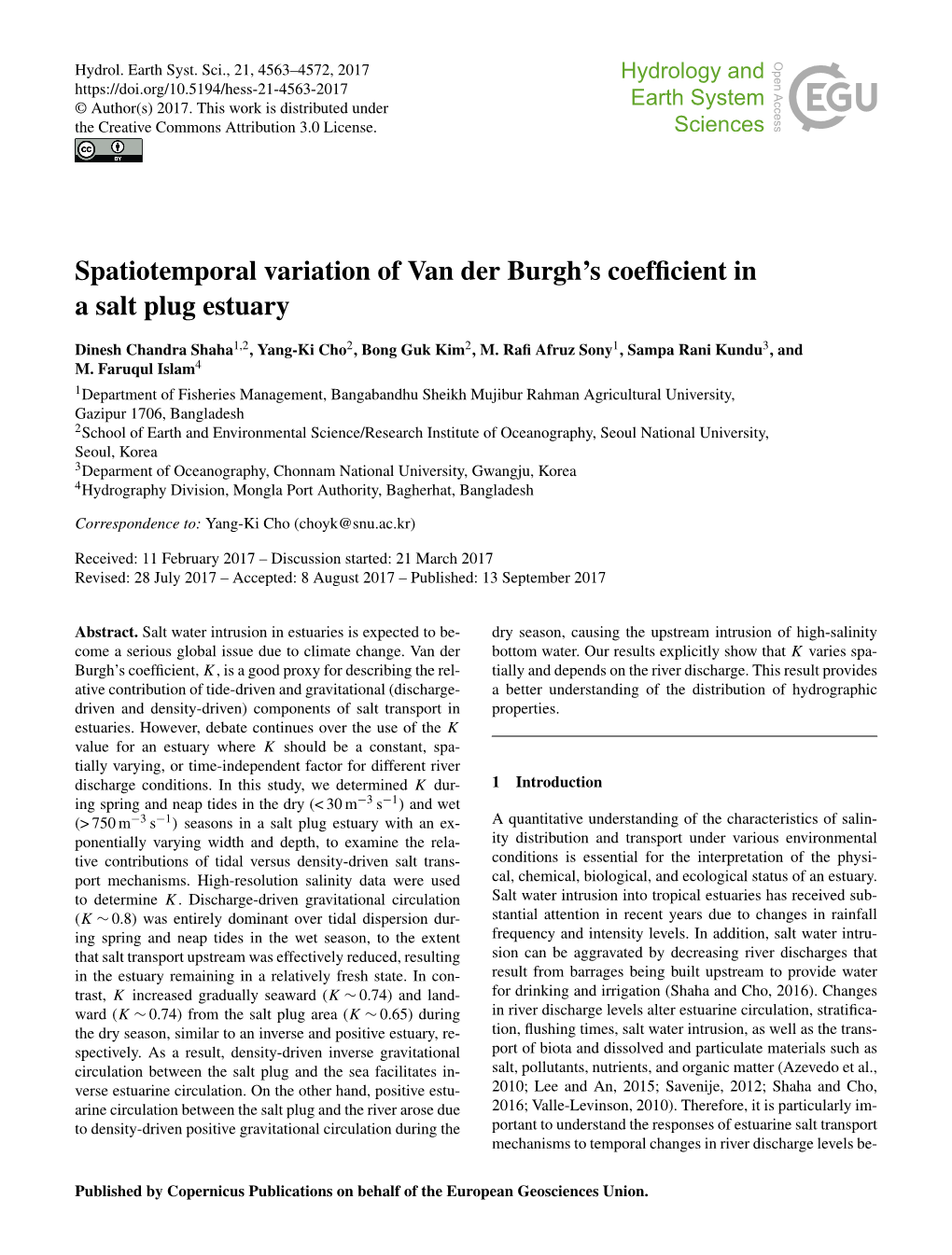
Load more
Recommended publications
-
Status of Fish and Shellfish Diversity and Their Decline Factors in the Rupsa River of Khulna in Bangladesh Sazzad Arefin1, Mrityunjoy Kunda1, Md
Archives of Agriculture and Environmental Science 3(3): 232-239 (2018) https://doi.org/10.26832/24566632.2018.030304 This content is available online at AESA Archives of Agriculture and Environmental Science Journal homepage: www.aesacademy.org e-ISSN: 2456-6632 ORIGINAL RESEARCH ARTICLE Status of fish and shellfish diversity and their decline factors in the Rupsa River of Khulna in Bangladesh Sazzad Arefin1, Mrityunjoy Kunda1, Md. Jahidul Islam1, Debasish Pandit1* and Ahnaf Tausif Ul Haque2 1Department of Aquatic Resource Management, Sylhet Agricultural University, Sylhet-3100, BANGLADESH 2Department of Environmental Science and Management, North South University, Dhaka, BANGLADESH *Corresponding author’s E-mail: [email protected] ARTICLE HISTORY ABSTRACT Received: 13 August 2018 The study was aimed to find out the present status and causes of fish and shellfish diversity Revised received: 21 August 2018 reduction in the Rupsa River of Bangladesh. Studies were conducted for a period of 6 months Accepted: 26 August 2018 from July to December 2016. Focus group discussions (FGD), questionnaire interviews (QI) and key informant interviews (KII) were done to collect appropriate data from the local fishers and resource persons. A total of 62 species of fish and shellfish from 23 families were found in the river and 9 species disappeared in last 10 years. The species availability status was Keywords remarked in three categories and obtained as 14 species were commonly available, 28 species were moderately available and 20 species were rarely available. The highest percentage of Aquaculture Biodiversity fishes was catfishes (24.19%). There was a gradual reduction in the species diversity from Fishes and shellfishes previous 71 species to present 62 species with 12.68% declined by last 10 years. -
BMJ Open Is Committed to Open Peer Review. As Part of This Commitment We Make the Peer Review History of Every Article We Publish Publicly Available
BMJ Open: first published as 10.1136/bmjopen-2017-016745 on 1 September 2017. Downloaded from BMJ Open is committed to open peer review. As part of this commitment we make the peer review history of every article we publish publicly available. When an article is published we post the peer reviewers’ comments and the authors’ responses online. We also post the versions of the paper that were used during peer review. These are the versions that the peer review comments apply to. The versions of the paper that follow are the versions that were submitted during the peer review process. They are not the versions of record or the final published versions. They should not be cited or distributed as the published version of this manuscript. BMJ Open is an open access journal and the full, final, typeset and author-corrected version of record of the manuscript is available on our site with no access controls, subscription charges or pay- per-view fees (http://bmjopen.bmj.com). If you have any questions on BMJ Open’s open peer review process please email [email protected] http://bmjopen.bmj.com/ on September 26, 2021 by guest. Protected copyright. BMJ Open: first published as 10.1136/bmjopen-2017-016745 on 1 September 2017. Downloaded from BMJ Open Psychological distress and quality of life: Rationale and protocol of a prospective cohort study in a rural district in Bangladesh For peer review only Journal: BMJ Open Manuscript ID bmjopen-2017-016745 Article Type: Protocol Date Submitted by the Author: 16-Mar-2017 Complete List of Authors: -
34418-023: Southwest Area Integrated Water Resources
Semiannual Environmental Monitoring Report Project No. 34418-023 December 2018 Southwest Area Integrated Water Resources Planning and Management Project - Additional Financing Prepared by Bangladesh Water Development Board for the People’s Republic of Bangladesh and the Asian Development Bank. This Semiannual Environmental Monitoring Report is a document of the borrower. The views expressed herein do not necessarily represent those of ADB's Board of Directors, Management, or staff, and may be preliminary in nature. In preparing any country program or strategy, financing any project, or by making any designation of or reference to a particular territory or geographic area in this document, the Asian Development Bank does not intend to make any judgments as to the legal or other status of any territory or area. Semi-Annual Environmental Monitoring Report, SAIWRPMP-AF, July-December 2018 Bangladesh Water Development Board SEMI-ANNUAL ENVIRONMENTAL MONITORING REPORT [Period July – December 2018] FOR Southwest Area Integrated Water Resources Planning and Management Project- Additional Financing Project Number: GoB Project No. 5151 Full Country Name: Bangladesh Financed by: ADB and Government of Bangladesh Prepared by: Bangladesh Water Development Board, Under Ministry of Water Resources, Govt. of Bangladesh. For: Asian Development Bank December 2018 Page | i Table of Contents Table of Contents .......................................................................................................................................... ii Executive -
Flora and Faunal Resources and Ecosystem Conservation in the Sundarbans
International Journal of Agriculture Innovations and Research Volume 5, Issue 3, ISSN (Online) 2319-1473 Manuscript Processing Details (dd/mm/yyyy) : Received : 03/12/2016 | Accepted on : 10/12/2016 | Published : 22/12/2016 Flora and Faunal Resources and Ecosystem Conservation in the Sundarbans Dr. A.S.M. Helal Siddiqui Senior Research Officer, Mangrove Silviculture Division, Bangladesh Forest Research Institute, Ministry of Environment and Forest, Muzgunni, Khulna-9000, Bangladesh. [email protected] Abstract – The Sundarbans is the largest single tract passur ( Xylocarpus mekongensis ), goran ( Ceriops mangrove forest in the whole world. The Sundarbans has a decandra ), keora ( Sonneratia apetala ), amur (Amoora high and rich biodiversity value. There are lot of flora and cucullata ), baen (Avicennia officinalis ), kankra ( Bruguiera fauna here in the jungle. Biological diversity has three main sp .), shingra ( Cynometra ramiflora ), khalshi ( Aegiceras components such as species diversity, genetics diversity and corniculatum ), kirpa ( Lumnitzera racemosa ), golpata ecosystems diversity. Bangladesh is represented by 40% of the world true mangrove species. Bangladesh has lost 10% of (Nypa fruticans ), garjan ( Rhizophora mucronata ), dhundul the true mangrove species in recent years. Sundarbans (X. granatum )[22]-[26]. possesses a rich faunal diversity even after disappearance of a good number of interesting species. A total of 4 species of mammal, 2 species of birds, 1 species of reptile has extinct and 10 species of mammal, 11 species of birds, 16 species of reptile and 1 species of amphibian has endangered. In addition, 2 species of Sundarbans fish has critically endangered, 2 species have been showed endangered and 5 more species are vulnerable. The degradation of biological diversity is a global crisis. -
Download Paper
DISCOVERY OF A POTENTIAL SITE FOR COMMUNITY-BASED SUSTAINABLE ECOTOURISM IN THE SUNDARBANS RESERVE FORESTS, BANGLADESH Mohammad Zahirul Haque School of Environmental and Natural Resources Sciences Faculty of Science and Technology Universiti Kebangsaan Malaysia 43600 Bangi, Selangor, Malaysia and Bangladesh Forest Department Bono Bhaban, Agargaon Dhaka 1207, Bangladesh Email: [email protected] Mohammad Imam Hasan Reza Institute for Environment and Development (LESTARI) Universiti Kebangsaan Malaysia 43600 Bangi, Selangor, Malaysia Email: [email protected] Md. Mahmudul Alam Senior Lecturer School of Economics, Finance & Banking (SEFB) College of Business (COB) Universiti Utara Malaysia (UUM) 06010 UUM Sintok, Kedah, Malaysia Email: [email protected] Zahir Uddin Ahmed Bangladesh Forest Department Bono Bhaban, Agargaon Dhaka 1207, Bangladesh. Email: [email protected] Md. Wasiul Islam Forestry and Wood Technology Discipline Khulna University Khulna, Bangladesh Email: [email protected] Citation Reference: Haque, M.Z., Reza, M.I.H., Alam, M.M., Ahmed, Z.U., and Islam, M.W. 2016. Discovery of a Potential Site for Community-based Sustainable Ecotourism in the Sundarbans Reserve Forests, Bangladesh. International Journal of Conservation Science, 7(2): 553- 566. http://www.ijcs.uaic.ro/public/IJCS-16-30_Haque.pdf This is a pre-publication copy. The published article is copyrighted by the publisher of the journal. 1 DISCOVERY OF A POTENTIAL SITE FOR COMMUNITY-BASED SUSTAINABLE ECOTOURISM IN THE SUNDARBANS RESERVE FORESTS, BANGLADESH Abstract Conservation of biological diversity is a pressing need and protected areas are the cornerstones for conserving remaining flora and fauna. However, forest dependent livelihood in countries like Bangladesh making this task very critical. In the case of the poor and forest-dependent livelihood in the Sundarbans area of Bangladesh, an eco-friendly ecotourism may provide an alternative livelihood, which may reduce the overexploitation from the valuable Sundarbans forest ecosystems. -
(Hamilton, 1822) in a Wetland Ecosystem (Beel Dakatia), Southwestern Bangladesh
Egyptian Journal of Aquatic Biology & Fisheries Zoology Department, Faculty of Science, Ain Shams University, Cairo, Egypt. ISSN 1110 – 6131 Vol. 25(3): 505 – 524 (2021) www.ejabf.journals.ekb.eg Growth, Condition, Maturity and Mortality of the Dwarf Gourami, Trichogaster lalius (Hamilton, 1822) in a Wetland Ecosystem (Beel Dakatia), Southwestern Bangladesh Newton Saha1*, Prosun Roy2, Zubyda Mushtari Nadia3, Wasim Akram4, Ferdous Ahamed1 5 and Md. Yeamin Hossain 1Department of Fisheries Management, Patuakhali Science and Technology University, Bangladesh 2Department of Aquaculture, Bangladesh Agricultural University, Bangladesh 3Department of Aquatic Animal Health Management, Sher-e-Bangla Agricultural University, Bangladesh 4Department of Fish Genetics and Biotechnology, Khulna University, Khulna-9208, Bangladesh 5Department of Fisheries, Faculty of Agriculture, University of Rajshahi, Bangladesh *Corresponding Author: [email protected] ARTICLE INFO ABSTRACT Article History: The dwarf gourami, Trichogaster lalius, is a low-cost freshwater edible fish Received: Feb. 4, 2021 for the people of South Asian countries containing a variety of essential Accepted: April. 11, 2021 nutrients. The first wide-ranging explanation on the population parameters Online: June 18, 2021 specifically; length-frequency distribution (LFD), length-weight relationships _______________ (LWRs), length-length relationship (LLR), form factor (a3.0), condition factors (allometric, K ; Fulton’s, K ; relative, K ; relative weight, W ), size at first Keywords: A F R R sexual maturity (L ) and natural mortality (M ) of T. lalius were estimated. 768 Population parameter, m w specimens were collected over one calendar year (March 2019 to February Beel Dakatia, 2020) from a wetland ecosystem named Beel Dakatia, Khulna, southwestern Trichogaster lalius, Bangladesh through various traditional fishing gears. -
List of 100 Bed Hospital
List of 100 Bed Hospital No. of Sl.No. Organization Name Division District Upazila Bed 1 Barguna District Hospital Barisal Barguna Barguna Sadar 100 2 Barisal General Hospital Barisal Barishal Barisal Sadar (kotwali) 100 3 Bhola District Hospital Barisal Bhola Bhola Sadar 100 4 Jhalokathi District Hospital Barisal Jhalokati Jhalokati Sadar 100 5 Pirojpur District Hospital Barisal Pirojpur Pirojpur Sadar 100 6 Bandarban District Hospital Chittagong Bandarban Bandarban Sadar 100 7 Comilla General Hospital Chittagong Cumilla Comilla Adarsha Sadar 100 8 Khagrachari District Hospital Chittagong Khagrachhari Khagrachhari Sadar 100 9 Lakshmipur District Hospital Chittagong Lakshmipur Lakshmipur Sadar 100 10 Rangamati General Hospital Chittagong Rangamati Rangamati Sadar Up 100 11 Faridpur General Hospital Dhaka Faridpur Faridpur Sadar 100 12 Madaripur District Hospital Dhaka Madaripur Madaripur Sadar 100 13 Narayanganj General (Victoria) Hospital Dhaka Narayanganj Narayanganj Sadar 100 14 Narsingdi District Hospital Dhaka Narsingdi Narsingdi Sadar 100 15 Rajbari District Hospital Dhaka Rajbari Rajbari Sadar 100 16 Shariatpur District Hospital Dhaka Shariatpur Shariatpur Sadar 100 17 Bagerhat District Hospital Khulna Bagerhat Bagerhat Sadar 100 18 Chuadanga District Hospital Khulna Chuadanga Chuadanga Sadar 100 19 Jhenaidah District Hospital Khulna Jhenaidah Jhenaidah Sadar 100 20 Narail District Hospital Khulna Narail Narail Sadar 100 21 Satkhira District Hospital Khulna Satkhira Satkhira Sadar 100 22 Netrokona District Hospital Mymensingh Netrakona -
Asian Journal of Medical and Biological Research Socio
Asian J. Med. Biol. Res. 2021, 7 (2), 164-173; doi: 10.3329/ajmbr.v7i2.54996 Asian Journal of Medical and Biological Research ISSN 2411-4472 (Print) 2412-5571 (Online) www.ebupress.com/journal/ajmbr Article Socio-economic status of fisher communities in Dengar beel under Melandah Upazila, Jamalpur, Bangladesh Md. Fakhrul Islam*, Syed Ariful Haque, Md. Saiful Islam, Partha Sarathi Das and Mizanur Rahman Department of Fisheries, Bangamata Sheikh Fojilatunnesa Mujib Science and Technology University, Melandah, Jamalpur-2012, Bangladesh *Corresponding author: Md. Fakhrul Islam, Department of Fisheries, Bangamata Sheikh Fojilatunnesa Mujib Science and Technology University, Melandah, Jamalpur, Bangladesh. Phone: +8801825446384; E-mail: [email protected] Received: 23 May 2021/Accepted: 19 June 2021/ Published: 30 June 2021 Abstract: The current study was conducted to evaluate the socio-economic profile of fisher communities in Dengar beel under Melandah Upazila of Jamalpur district, Bangladesh. A total of 45 fishers were surveyed from November 2020 to April 2021. It was found that the family size of 53% of fishers was medium, consisting of 5- 6 members. Most of the fisher communities belonged to the age group above 45 years old, represented by 100% Muslim. About 73% of fishers were less educated, 13% primary level, 7% secondary level, and 7% Secondary School Certificate passed and above, respectively. Most of the fishers (60%) have katcha houses with tin roofing, 7% have katcha house with straw roofs, 13% have semi- pucca houses and 20% have pucca houses. Around 93% of fishers used their own tube-well while only 7% used neighbours’ tube-well. All of the fisher household were connected with electricity supply. -
Situation Assessment Report in S-W Coastal Region of Bangladesh
Livelihood Adaptation to Climate Change Project (BGD/01/004/01/99) SITUATION ASSESSMENT REPORT IN S-W COASTAL REGION OF BANGLADESH (JUNE, 2009) Food and Agriculture Organization of the United Nations (FAO) Department of Agricultural Extension (DAE) Acknowledgements The present study on livelihoods adaptation was conducted under the project Livelihood Adaptation to Climate Change, project phase-II (LACC-II), a sub-component of the Comprehensive Disaster Management Programme (CDMP), funded by UNDP, EU and DFID which is being implemented by the Department of Agricultural Extension (DAE) with technical support of the Food and Agriculture Organization (FAO), UN. The Project Management Unit is especially thankful to Dr Stephan Baas, Lead Technical Advisor (Environment, Climate Change and Bioenergy Division (NRC), FAO, Rome) and Dr Ramasamy Selvaraju, Environment Officer (NRC Division, FAO, Rome) for their overall technical guidance and highly proactive initiatives. The final document and the development of the project outputs are direct results of their valuable insights received on a regular basis. The inputs in the form of valuable information provided by Field Officers (Monitoring) of four coastal Upazilas proved very useful in compiling the report. The reports of the upazilas are very informative and well presented. In the course of the study, the discussions with a number of DAE officials at central and field level were found insightful. In devising the fieldwork the useful contributions from the DAE field offices in four study upazilas and in district offices of Khulna and Pirojpur was significant. The cooperation with the responsible SAAOs in four upazilas was also highly useful. The finalization of the study report has benefited from the valuable inputs, comments and suggestions received from various agencies such as DAE, Climate Change Cell, SRDI (Central and Regional offices), and others. -
Heavy Metals Contamination of River Water and Sediments in the Mangrove Forest Ecosystems in Bangladesh: a Consequence of Oil Spill Incident
Heavy Metals Contamination of River Water and Sediments in The Mangrove Forest Ecosystems in Bangladesh: A Consequence of Oil Spill Incident Tasrina Rabia Choudhury ( [email protected] ) Bangladesh Atomic Energy Commission https://orcid.org/0000-0001-6717-2550 Thamina Acter East-West University Nizam Uddin Daffodil International University Masud Kamal Bangladesh Atomic Energy Commission A.M. Sarwaruddin Chowdhury Dhaka University M. Saur Rahman Bangladesh Atomic Energy Commission Research Article Keywords: Sundarbans, lead, chromium, water quality index, metal pollution index, enrichment factor, geo-accumulation Posted Date: February 15th, 2021 DOI: https://doi.org/10.21203/rs.3.rs-164143/v1 License: This work is licensed under a Creative Commons Attribution 4.0 International License. Read Full License Page 1/24 Abstract Oil spillage is one of the common pollution events of global water-soil ecosystems. A comprehensive investigation on heavy metals pollution of surface water and sediments was conducted after oil spill incident in Sela River and its tributaries of the Sundarbans mangrove forest ecosystems, Bangladesh. Water and sediment samples were collected from the preselected sampling points in Sela River, and the elemental (Pb, Cd, Cr, Co, Cu, Ni, Fe, As, Hg, Mn, Zn, Ca, Mg, Na, and K) analysis was done using atomic absorption spectrometer (AAS). This study revealed that the descending order for the average concentration of the studied elements were found to be Mg > Co > Na > Ni > K > Ca > Pb > Fe > Mn > Cr > Cd > Zn > Cu respectively, while As and Hg in water samples were found to be below detection limit (BDL). However, some of the toxic elements in the Sela River water samples were exceeded the permissible limit set by the World Health Organization (WHO) with a descending order of Co > Cd > Pb > Ni respectively. -
Qualitative Evaluation of Food for Peace Development Food Assistance Projects in Bangladesh
Qualitative Evaluation of Food for Peace Development Food Assistance Projects in Bangladesh F. James Levinson Jessica Blankenship Julian Francis Kusum Hachhethu Rezaul Karim Kathleen Kurz Nashida Akbar Maqbul Bhuiyan May 2016 This report is made possible by the generous support Recommended Citation of the American people through the support of the Levinson, F. James; Blankenship, Jessica; Francis, Office of Health, Infectious Diseases, and Nutrition, Julian; Hachhethu, Kusum; Karim, Rezaul; Kurz, Bureau for Global Health, and the Office of Food for Kathleen; Akbar, Nashida; and Bhuiyan, Maqbul. Peace, Bureau for Democracy, Conflict, and 2016. Qualitative Evaluation of Food for Peace Humanitarian Assistance, U.S. Agency for Development Food Assistance Projects in International Development (USAID) under terms of Bangladesh. Washington, DC: FHI 360/Food and Cooperative Agreement No. AID-OAA-A-12-00005, Nutrition Technical Assistance III Project (FANTA). through the Food and Nutrition Technical Assistance III Project (FANTA), managed by FHI 360. Contact Information The contents are the responsibility of FHI 360 and do Food and Nutrition Technical Assistance III Project not necessarily reflect the views of USAID or the (FANTA) United States Government. FHI 360 1825 Connecticut Avenue, NW May 2016 Washington, DC 20009-5721 T 202-884-8000 F 202-884-8432 [email protected] www.fantaproject.org Qualitative Evaluation of Food for Peace Development Food Assistance Projects in Bangladesh Acknowledgments The authors would like to thank Diana Stukel, Kavita Sethuraman, Reena Borwankar, Megan Deitchler, and the superb FANTA Communications team for their continual encouragement and creative suggestions. We would also like to acknowledge our USAID colleagues in Dhaka and in Washington. -
Pre-Disaster Secondary Data
PRE-DISASTER SECONDARY DATA Date of Publication: March 2014 Prepared by: The JNA Consolidation Project, Bangladesh (supported by ACAPS) Nature of disaster: Waterlogging Note about this document: This secondary data review was undertaken in Bangladesh with the support of Government, NGOs, and UN agencies. The document has been reviewed by each of the clusters to ensure accuracy. This is a living document and will be updated on a regular basis, as new information becomes available. Feedback on the document should be made to ACAPS at [email protected]. Word versions of this document are available from ACAPS. COUNTRY PROFILE CLIMATE AND GEOGRAPHY Disaggregated pre-crisis demographic and socio-economic data from the 2011 Census is available on the Humanitarian Country Task Team’s web platform Bangladesh is 147,570 km² and consists of a flood plain made up of the Ganges, (HCTT). Brahmaputra, and Meghna rivers flowing into the Bay of Bengal. Population density is 1,014 people/km², compared 411 in India, 189 in Nepal, and The river delta comprises over 230 rivers and tributaries. 119 in France (WB Indicators) Two thirds of its land areas is <5 metres below sea level, however, the southeast 39.7% of the population is <18 years (CEA 2013). is hilly (WB 2011). 7% of the population is over the age of 60, of those 11% is over 80 (UNDESA 2012). The average temperature in the cooler months is 12-25° C (November – May) and The average household size is 4.4 persons (urban 4.4 and rural 4.3) (Census 2011). is 23-35° C (June – September).