Searches for Exotic Radio Sources and Intelligent Life on Other Worlds
Total Page:16
File Type:pdf, Size:1020Kb
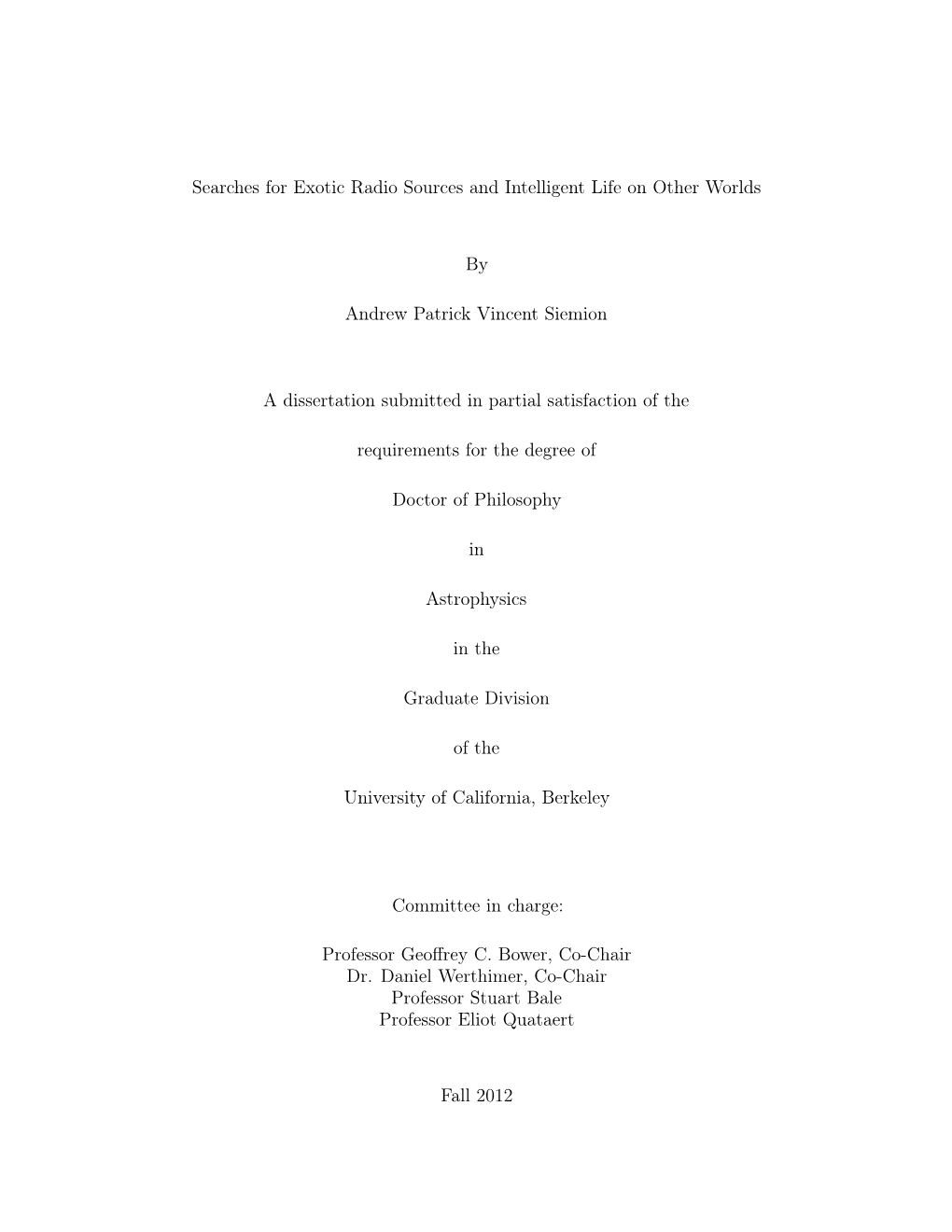
Load more
Recommended publications
-
CASKAR: a CASPER Concept for the SKA Phase 1 Signal Processing Sub-System
CASKAR: A CASPER concept for the SKA phase 1 Signal Processing Sub-system Francois Kapp, SKA SA Outline • Background • Technical – Architecture – Power • Cost • Schedule • Challenges/Risks • Conclusions Background CASPER Technology MeerKAT Who is CASPER? • Berkeley Wireless Research Center • Nancay Observatory • UC Berkeley Radio Astronomy Lab • Oxford University Astrophysics • UC Berkeley Space Sciences Lab • Metsähovi Radio Observatory, Helsinki University of • Karoo Array Telescope / SKA - SA Technology • NRAO - Green Bank • New Jersey Institute of Technology • NRAO - Socorro • West Virginia University Department of Physics • Allen Telescope Array • University of Iowa Department of Astronomy and • MIT Haystack Observatory Physics • Harvard-Smithsonian Center for Astrophysics • Ohio State University Electroscience Lab • Caltech • Hong Kong University Department of Electrical and Electronic Engineering • Cornell University • Hartebeesthoek Radio Astronomy Observatory • NAIC - Arecibo Observatory • INAF - Istituto di Radioastronomia, Northern Cross • UC Berkeley - Leuschner Observatory Radiotelescope • Giant Metrewave Radio Telescope • University of Manchester, Jodrell Bank Centre for • Institute of Astronomy and Astrophysics, Academia Sinica Astrophysics • National Astronomical Observatories, Chinese Academy of • Submillimeter Array Sciences • NRAO - Tucson / University of Arizona Department of • CSIRO - Australia Telescope National Facility Astronomy • Parkes Observatory • Center for Astrophysics and Supercomputing, Swinburne University -
Russell Tracy Crawford Papers MS.265
http://oac.cdlib.org/findaid/ark:/13030/c8sf31rj No online items Guide to the Russell Tracy Crawford papers MS.265 Maureen Carey and Alix Norton University of California, Santa Cruz 2016 1156 High Street Santa Cruz 95064 [email protected] URL: http://guides.library.ucsc.edu/speccoll Guide to the Russell Tracy MS.265 1 Crawford papers MS.265 Language of Material: English Contributing Institution: University of California, Santa Cruz Title: Russell Tracy Crawford papers creator: Crawford, R. T. (Russell Tracy), 1876- Identifier/Call Number: MS.265 Physical Description: 2.45 Linear Feet3 boxes, 1 oversize box Date (inclusive): 1897-1960 Access Collection is open for research. Arrangement This collection is organized into five series: 1. Biographical 2. Correspondence 3. Notes and notebooks 4. Observations and charts 5. Writings Materials within each series are arranged in chronological order. Biographical / Historical Russell Tracy Crawford was an astronomer and professor who was associated with the University of California for over 50 years. Earning his degrees in astronomy in 1897 and 1901, he then worked as a professor and astronomer for the university in various capacities until his retirement in 1946. Crawford earned one of the first fellowships granted by the Lick Observatory in 1897, and finished his doctoral work there using the meridian circle with astronomer R.H. Tucker. Crawford is well known for his work in theoretical astronomy, and his observations and computations of comet orbits. He published over a hundred articles and a book entitled The Determination of Orbits of Comets and Asteroids. Crawford was born on March 26, 1876, in Davis, California. -
BV RI Photometry of Supernovae
View metadata, citation and similar papers at core.ac.uk brought to you by CORE provided by CERN Document Server Accepted by Publications of the Astronomical Society of the Pacific BV RI Photometry of Supernovae Wynn C. G. Ho1;2, Schuyler D. Van Dyk3,ChienY.Peng4, Alexei V. Filippenko5, Douglas C. Leonard6, Thomas Matheson7, Richard R. Treffers8 Department of Astronomy, University of California, Berkeley, CA 94720-3411 and Michael W. Richmond9 Department of Physics, Rochester Institute of Technology, Rochester, NY 14623-5603 ABSTRACT We present optical photometry of one Type IIn supernova (1994Y) and nine Type Ia super- novae (1993Y, 1993Z, 1993ae, 1994B, 1994C, 1994M, 1994Q, 1994ae, and 1995D). SN 1993Y and SN 1993Z appear to be normal SN Ia events with similar rates of decline, but we do not have data near maximum brightness. The colors of SN 1994C suggest that it suffers from significant reddening or is intrinsically red. The light curves of SN 1994Y are complicated; they show a slow rise and gradual decline near maximum brightness in VRI and numerous changes in the decline rates at later times. SN 1994Y also demonstrates color evolution similar to that of the SN IIn 1988Z, but it is slightly more luminous and declines more rapidly than SN 1988Z. The be- havior of SN 1994Y indicates a small ejecta mass and a gradual strengthening of the Hα emission relative to the continuum. Subject headings: supernovae: general – supernovae: individual (1993Y, 1993Z, 1993ae, 1994B, 1994C, 1994M, 1994Q, 1994Y, 1994ae, 1995D) 1Also associated with Space Sciences Laboratory, and Department of Physics, University of California, Berkeley. -
Looking for Life at Leuschner
LAMORINDA WEEKLY | Looking for Life at Leuschner Published July 29th, 2015 Looking for Life at Leuschner By Cathy Dausman Many Moraga residents can easily point out the Saint Mary's College hillside observatory. But like a distant star nearly invisible to the naked eye, another observatory sits largely unnoticed among the hills of Lafayette - the Leuschner Observatory that belongs to UC Berkeley. The Leuschner site, adjacent to Briones Regional Park and East Bay Municipal Utility District watershed land, is comprised of two domed buildings. The first holds a 30-inch optical telescope used by astronomy students from UC Berkeley and San Francisco State University. The smaller domed building housing a 20-inch telescope is currently off limits to human occupancy because of its rodent population. "We haven't been in there for decades," said UC Berkeley Astronomy Department Chair Imke de Pater. As rough around the edges as it presently is, Leuschner is still a far sight better than what Cal astronomy students used to rely on. In 1936, the only practical student lab was a small dome atop UC's Campbell Hall. But Campbell Hall's "students' observatory," as it was then called, wasn't cutting it - there was simply too much light and radio wave interference in an urban location. In the early 1960s the Russell family deeded a 283-acre parcel of Lafayette land to Berkeley's College of Natural Resources. It was named the Russell Research Station and was to be used for Inside the Leuschner Observatory in wild land and forestry research. Within the acreage Lafayette Photos Cathy Dausman was a 900-plus-foot hilltop above the fog line and away from light pollution - a far better site for an observatory. -
Lick Observatory Records: Photographs UA.036.Ser.07
http://oac.cdlib.org/findaid/ark:/13030/c81z4932 Online items available Lick Observatory Records: Photographs UA.036.Ser.07 Kate Dundon, Alix Norton, Maureen Carey, Christine Turk, Alex Moore University of California, Santa Cruz 2016 1156 High Street Santa Cruz 95064 [email protected] URL: http://guides.library.ucsc.edu/speccoll Lick Observatory Records: UA.036.Ser.07 1 Photographs UA.036.Ser.07 Contributing Institution: University of California, Santa Cruz Title: Lick Observatory Records: Photographs Creator: Lick Observatory Identifier/Call Number: UA.036.Ser.07 Physical Description: 101.62 Linear Feet127 boxes Date (inclusive): circa 1870-2002 Language of Material: English . https://n2t.net/ark:/38305/f19c6wg4 Conditions Governing Access Collection is open for research. Conditions Governing Use Property rights for this collection reside with the University of California. Literary rights, including copyright, are retained by the creators and their heirs. The publication or use of any work protected by copyright beyond that allowed by fair use for research or educational purposes requires written permission from the copyright owner. Responsibility for obtaining permissions, and for any use rests exclusively with the user. Preferred Citation Lick Observatory Records: Photographs. UA36 Ser.7. Special Collections and Archives, University Library, University of California, Santa Cruz. Alternative Format Available Images from this collection are available through UCSC Library Digital Collections. Historical note These photographs were produced or collected by Lick observatory staff and faculty, as well as UCSC Library personnel. Many of the early photographs of the major instruments and Observatory buildings were taken by Henry E. Matthews, who served as secretary to the Lick Trust during the planning and construction of the Observatory. -
Jill Tarter PUBLIC LECTURE TRANSCRIPT
Jill Tarter PUBLIC LECTURE TRANSCRIPT March 3, 2015 KANE HALL 130 | 7:30 P.M. TABLE OF CONTENTS INTRODUCTION, page 1 Marie Clement, Graduate Student, Chemistry FEATURED SPEAKER, page 1 Jill Tarter, Bernard M. Oliver chair for SETI Q&A SESSION, page 8 OFFICE OF PUBLIC LECTURES So our speaker tonight is Dr. Jill Cornell Tarter. Jill Tarter holds INTRODUCTION the Bernard M. Oliver chair for SETI, the Search for Extraterrestrial Intelligence at the SETI Institute in Mountain View, California. Tarter received her Bachelor of Engineering Physics degree with distinction from Cornell University and her Marie Clement master's degree and Ph.D. in astronomy from the University of California Berkeley. She served as project scientist for NASA Graduate Student SETI program, the High Resolution Microwave Survey, and has conducted numerous observational programs at radio Good evening, and welcome to tonight's Jessie and John Danz observatories worldwide. Since the termination of funding for endowed public lecture with Jill Cornell Tarter. I am Marie NASA SETI program in 1993, she has served in a leadership role Clement, a graduate student in the chemistry department and a to secure private funding to continue this exploratory science. member of the student organization, Women in Chemical Tarter’s work has brought her wide recognition in the scientific Sciences. Before we introduce tonight's speaker, I want to community, including the Lifetime Achievement Award from share some background about the generous gift to the Women in Aerospace, two Public Service Medals from NASA, University of Washington that allows the Graduate School to Chabot Observatory’s Person of the Year award, Women of host the series: the Jessie and John Danz Endowment. -
Upcoming Events: Science@Cal Monthly Lectures 3Rd Saturday of Each Month 11:00 A.M
University of California, Berkeley Department of Astronomy B E R K E L E Y A S T R O N O M Y Hearst Field Annex MC 3411 Berkeley, CA 94720-3411 Upcoming Events: Science@Cal Monthly Lectures 3rd Saturday of each month 11:00 a.m. UC Berkeley Campus location changes each month Consult website for details http://scienceatcal. berkeley.edu/lectures UniversityUNIVERSITY of California OF CALIFORNIA | 2014 2014 Evening with the Stars TBA Fall 2014 From the Chair’s Desk… co-PI on the founding grant for the Berkeley the Friends of Astrophysics Postdoctoral Please see the Astronomy website for more Institute for Data Science (BIDS) to bring Fellowship and many other coveted prize information together scientists with diverse backgrounds, fellowships. http://astro.berkeley.edu The Astronomy Department is a yet all dealing with “Big Data” (see page In addition to the rigorous research they vibrant, evolving 2). He is also on the management council pursue, our students and postdocs also find 2015 Raymond and Beverly Sackler community. It’s hard that oversees the Large Synoptic Survey time to get involved in other interesting Distinguished Lecture in Astronomy to fully capture all Telescope. This past summer Bloom again career-building and public outreach activities. Carolyn Porco, Space Science Institute that is happening organized the annual Python Language Matt George, Adam Morgan, and Chris Klein Public lecture: Wednesday, Jan 28 here in a few brief Bootcamp, an immensely popular three-day joined the Insight Data Science Fellows Joint Astronomy/Earth Planetary Sciences paragraphs, but I’ll summer workshop with >200 attendees. -
An Infrared Camera for Leuschner Observatory and the Berkeley
An Infrared Camera for Leuschner Observatory and the Berkeley Undergraduate Astronomy Lab James R. Graham and Richard R. Treffers Astronomy Department, University of California, Berkeley, CA 94720-3411 jrg, [email protected] ABSTRACT We describe the design, fabrication, and operation of an infrared camera which is in use at the 30-inch telescope of the Leuschner Observatory. The camera is based on a Rockwell PICNIC 256×256 pixel HgCdTe array, which is sensitive from 0.9-2.5 µm. The primary purpose of this telescope is for undergraduate instruction. The cost of the camera has been minimized by using commercial parts whereever practical. The camera optics are based on a modified Offner relay which forms a cold pupil where stray thermal radiation from the telescope is baffled. A cold, six-position filter wheel is driven by a cryogenic stepper motor, thus avoiding any mechanical feed throughs. The array control and readout electronics are based on standard PC cards; the only custom component is a simple interface card which buffers the clocks and amplifies the analog signals from the array. Submitted to Publications of the Astronomical Society of the Pacific: 2001 Jan 10, Accepted 2001 Jan 19 Subject headings: instrumentation: detectors, photometers 1. Introduction astronomy labs offer a potent antidote to conven- tional lectures, and stimulate students to pursue Many undergraduates enter universities and the astronomy major. We describe a state-of-the- colleges with the ambition of pursing several sci- art infrared camera which is used at Berkeley to ence courses, or even a science major. Yet sig- inspire and instruct. -
Astronomy (ASTRON) 1
Astronomy (ASTRON) 1 ASTRON 7B Introduction to Astrophysics 4 Astronomy (ASTRON) Units Terms offered: Spring 2021, Spring 2020, Spring 2019 Courses This is the second part of an overview of astrophysics, which begins with 7A. This course covers the Milky Way galaxy, star formation and Expand all course descriptions [+]Collapse all course descriptions [-] the interstellar medium, galaxies, black holes, quasars, dark matter, the ASTRON 3 Introduction to Modern expansion of the universe and its large-scale structure, and cosmology Cosmology 2 Units and the Big Bang. The physics in this course includes that used in Terms offered: Fall 2015, Spring 2015, Spring 2014 7A (mechanics and gravitation; kinetic theory of gases; properties of Description of research and results in modern extragalactic astronomy radiation and radiative energy transport; quantum mechanics of photons, and cosmology. We read the stories of discoveries of the principles of our atoms, and electrons; and magnetic fields) and adds the special and Universe. Simple algebra is used. general theories of relativity. Introduction to Modern Cosmology: Read More [+] Introduction to Astrophysics: Read More [+] Hours & Format Rules & Requirements Fall and/or spring: 15 weeks - 2 hours of lecture per week Prerequisites: Math 1A -1B. Physics 5A, 5B/5BL, 5C/5CL, or Physics 7A/B/C (5C or 7C can be taken concurrently) Additional Details Hours & Format Subject/Course Level: Astronomy/Undergraduate Fall and/or spring: 15 weeks - 3 hours of lecture and 1 hour of Grading/Final exam status: Letter grade. Final exam required. laboratory per week Instructors: Bloom, Ma Additional Details Introduction to Modern Cosmology: Read Less [-] Subject/Course Level: Astronomy/Undergraduate ASTRON 7A Introduction to Astrophysics 4 Grading/Final exam status: Letter grade. -
Lawrence Berkeley Laboratory UNIVERSITY of CALIFORNIA
Lawrence Berkeley National Laboratory Recent Work Title AN AUTOMATED SEARCH FOR SUPERNOVA EXPLOSIONS Permalink https://escholarship.org/uc/item/4nm5z2q7 Author Kare, J.T. Publication Date 1987-07-01 eScholarship.org Powered by the California Digital Library University of California LBL-23782 Preprint Lawrence Berkeley Laboratory UNIVERSITY OF CALIFORNIA Physics Division RC:CEIVED LP.WRE:\.ICE BER~ELEVLA80RATORY SEP181987 LIBRARY AND DOCUMEI\ITS SECTION Submitted to Review of Scientific Instruments An Automated Search for Supernova Explosions J.T. Kare, M.S. Burns, F.S. Crawford, P.G. Friedman, R.A. Muller, C.R. Pennypacker, S. Perlmutter, R. Treffers, and R. Williams --. ~ -- -----~~~--- -- --- July 1987 i I ! TWO-WEEK LOAN COPY i This is a Library Circulating Copy ·' 1 which may" be borrowed for .two w~ek~~' ~~~~ --- ..... Prepared for the U.S. Department of Energy under Contract DE-AC03-76SF00098 DISCLAIMER This document was prepared as an account of work sponsored by the United States Government. While this document is believed to contain correct information, neither the United States Government nor any agency thereof, nor the Regents of the University of California, nor any of their employees, makes any warranty, express or implied, or assumes any legal responsibility for the accuracy, completeness, or usefulness of any information, apparatus, product, or process disclosed, or represents that its use would not infringe privately owned rights. Reference herein to any specific commercial product, process, or service by its trade name, trademark, manufacturer, or otherwise, does not necessarily constitute or imply its endorsement, recommendation, or favoring by the United States Government or any agency thereof, or the Regents of the University of California. -
Searching for ETI Ragbir Bhathal
Journal & Proceedings of the Royal Society of New South Wales, Vol. 141, p. 33–37, 2008 ISSN 0035-9173/08/020033–5 $4.00/1 Searching for ETI ragbir bhathal Abstract: The search for extraterrestrial intelligence is a scientific experiment which has been pursued for the last forty-five years. Over one hundred searches (ranging from one-off to sporadic and continuous) in both the microwave and optical regions of the electromagnetic spectrum have been carried out during this time. To date no ETI signals with the required signature have been discovered. This paper discusses some of the most significant searches and future directions in the search for extraterrestrial intelligence. Keywords: ETI search strategies; ETI radio searches, Nanosecond pulses. INTRODUCTION on so called ‘magic’ frequencies (the 21 cm hy- drogen line) with frequency resolutions varying The scientific search for extraterrestrial intelli- from Megahertz to a few hundredths of a Hertz. gence or seti for short had its beginnings in The size of the radio telescopes has ranged the second half of the 20th century. In its from a few metres in diameter to the giant 305 early years the seti program was plagued by metres instrument at Arecebo, the world’s most questions of its validity as a scientific discipline. sensitive and largest radio telescope. In fact, several members of the IAU Commission About ten years after Project Ozma the first 51 were extremely critical of it. However, this dedicated search in the USA for ETI signals was has not stopped astronomers and astrobiologists carried out under the direction of J. -
2016 Newsletter
1 BERKELEY ASTRONOMY UNIVERSITY OF CALIFORNIA AT BERKELEY WINTER 2016 Astronomy in the News For full versions of news articles, visit news.berkeley.edu CONTENTS Astromony in the News ...................1 SUPERMASSIVE BLACK LONG-TERM, HI-RES Chair’s Message ................................4 HOLES MAY BE LURKING TRACKING OF ERUPTIONS Getting to know new faculty ..........4 EVERYWHERE IN THE ON JUPITER’S MOON IO Faculty awards and highlights ....... 5 UNIVERSE OCTOBER 20, 2016–Bob Sanders, Media Retirements ........................................6 APRIL 6, 2016–Bob Sanders, Media Relations Relations Leuschner Observatory Update ...7 Evening with the Stars .....................7 A near-record supermassive black hole Jupiter’s moon Io continues to be the most discovered in a sparse area of the local volcanically active body in the solar system, Undergrad Symposium ...................7 universe indicate that these monster as documented by the longest series of Student Prizes and Awards ............8 objects — this one equal to 17 billion frequent, high-resolution observations of Sackler Lecture .................................9 suns — may be more common than the moon’s thermal emission ever obtained. In Memoriam .....................................9 once thought, according to UC Berkeley Using near-infrared adaptive optics on two Astro Nights .......................................9 astronomers. of the world’s largest telescopes — the Upcoming Events ...........................10 Until now, the biggest supermassive black 10-meter Keck II and the 8-meter Gemini Give to Astronomy .........................10 holes — those with masses at or near 10 North, both located near the summit of billion times that of our sun — have been the dormant volcano Maunakea in Hawaii — UC Berkeley astronomers tracked 48 over time, as if one triggered another 500 found at the cores of very large galaxies in kilometers away.