UNIVERSITY of CALGARY an Atomic Source of Quantum Light By
Total Page:16
File Type:pdf, Size:1020Kb
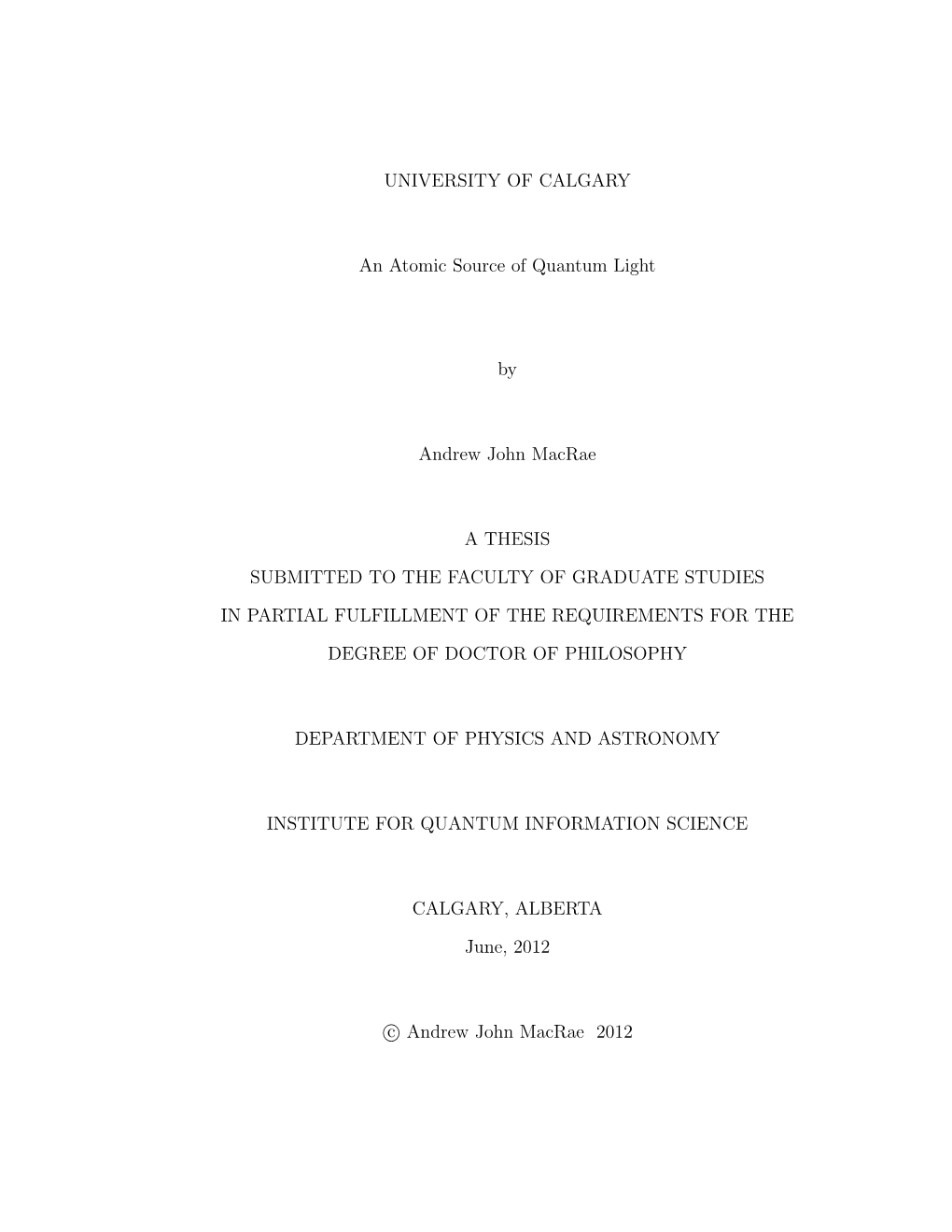
Load more
Recommended publications
-
Classical Models for Quantum Light
Classical Models for Quantum Light Arnold Neumaier Fakult¨atf¨urMathematik Universit¨atWien, Osterreich¨ Lecture given on April 7, 2016 at the Zentrum f¨urOberfl¨achen- und Nanoanalytik Johannes Kepler Universit¨atLinz, Osterreich¨ http://www.mat.univie.ac.at/~neum/papers/physpapers.html#CQlight 1 Abstract In this lecture, a timeline is traced from Huygens' wave optics to the modern concept of light according to quantum electrodynamics. The lecture highlights the closeness of classical concepts and quantum concepts to a surprising extent. For example, it is shown that the modern quantum concept of a qubit was already known in 1852 in fully classical terms. 2 A timeline of light 0: Prehistory I: Waves or particles? (1679{1801) II: Polarization (1809{1852) III: The electromagnetic field (1862{1887) IV: Shadows of new times coming (1887{1892) V: Energies are sometimes quantized (1900{1914) VI: The photon as unitary representation (1926{1946) VII: Quantum electrodynamics (1948{1949) VIII: Coherence and photodetection (1955{1964) IX: Stochastic and nonclassical light (1964-2004) I picked for each period only one particular theme. 3 A timeline of light 0: Prehistory In the beginning God created the heavens and the earth. And God said, "Let there be light", and there was light. (Genesis 1:1.3) It took him only 10 seconds... 10s after the Big Bang: photon epoch begins 2134 BC: oldest solar eclipse recorded in human history (2 Chinese astronomers lost their head for failing to predict it) ca. 1000 BC: It is the glory of God to conceal things, but the kings' pride is to research them. -
`Nonclassical' States in Quantum Optics: a `Squeezed' Review of the First 75 Years
Home Search Collections Journals About Contact us My IOPscience `Nonclassical' states in quantum optics: a `squeezed' review of the first 75 years This article has been downloaded from IOPscience. Please scroll down to see the full text article. 2002 J. Opt. B: Quantum Semiclass. Opt. 4 R1 (http://iopscience.iop.org/1464-4266/4/1/201) View the table of contents for this issue, or go to the journal homepage for more Download details: IP Address: 132.206.92.227 The article was downloaded on 27/08/2013 at 15:04 Please note that terms and conditions apply. INSTITUTE OF PHYSICS PUBLISHING JOURNAL OF OPTICS B: QUANTUM AND SEMICLASSICAL OPTICS J. Opt. B: Quantum Semiclass. Opt. 4 (2002) R1–R33 PII: S1464-4266(02)31042-5 REVIEW ARTICLE ‘Nonclassical’ states in quantum optics: a ‘squeezed’ review of the first 75 years V V Dodonov1 Departamento de F´ısica, Universidade Federal de Sao˜ Carlos, Via Washington Luiz km 235, 13565-905 Sao˜ Carlos, SP, Brazil E-mail: [email protected] Received 21 November 2001 Published 8 January 2002 Online at stacks.iop.org/JOptB/4/R1 Abstract Seventy five years ago, three remarkable papers by Schrodinger,¨ Kennard and Darwin were published. They were devoted to the evolution of Gaussian wave packets for an oscillator, a free particle and a particle moving in uniform constant electric and magnetic fields. From the contemporary point of view, these packets can be considered as prototypes of the coherent and squeezed states, which are, in a sense, the cornerstones of modern quantum optics. Moreover, these states are frequently used in many other areas, from solid state physics to cosmology. -
An Atomic Source of Quantum Light
University of Calgary PRISM: University of Calgary's Digital Repository Graduate Studies The Vault: Electronic Theses and Dissertations 2012-10-25 An Atomic Source of Quantum Light MacRae, Andrew MacRae, A. (2012). An Atomic Source of Quantum Light (Unpublished doctoral thesis). University of Calgary, Calgary, AB. doi:10.11575/PRISM/24840 http://hdl.handle.net/11023/310 doctoral thesis University of Calgary graduate students retain copyright ownership and moral rights for their thesis. You may use this material in any way that is permitted by the Copyright Act or through licensing that has been assigned to the document. For uses that are not allowable under copyright legislation or licensing, you are required to seek permission. Downloaded from PRISM: https://prism.ucalgary.ca UNIVERSITY OF CALGARY An Atomic Source of Quantum Light by Andrew John MacRae A THESIS SUBMITTED TO THE FACULTY OF GRADUATE STUDIES IN PARTIAL FULFILLMENT OF THE REQUIREMENTS FOR THE DEGREE OF DOCTOR OF PHILOSOPHY DEPARTMENT OF PHYSICS AND ASTRONOMY INSTITUTE FOR QUANTUM INFORMATION SCIENCE CALGARY, ALBERTA October, 2012 �c Andrew John MacRae 2012 Abstract This thesis presents the experimental demonstration of an atomic source of narrowband nonclassical states of light. Employing four-wave mixing in hot atomic Rubidium vapour, the optical states produced are naturally compatible with atomic transitions and may be thus employed in atom-based quantum communication protocols. We first demonstrate the production of two-mode intensity-squeezed light and ana lyze the correlations between the two produced modes. Using homodyne detection in each mode, we verify the production of two-mode quadrature-squeezed light, achieving a reduction in quadrature variance of 3 dB below the standard quantum limit. -
Matthewthorntonphdthesis.Pdf (11.82Mb)
AGILE QUANTUM CRYPTOGRAPHY AND NON-CLASSICAL STATE GENERATION Matthew Thornton A Thesis Submitted for the Degree of PhD at the University of St Andrews 2020 Full metadata for this item is available in St Andrews Research Repository at: http://research-repository.st-andrews.ac.uk/ Please use this identifier to cite or link to this item: http://hdl.handle.net/10023/21361 This item is protected by original copyright Agile quantum cryptography and non-classical state generation Matthew Thornton This thesis is submitted in partial fulfilment for the degree of Doctor of Philosophy (PhD) at the University of St Andrews March 2020 PUBLICATIONS The following publications have resulted from the research contained in this Thesis: M. Thornton, H. Scott, C. Croal and N. Korolkova: Continuous-variable quantum digital signatures over insecure quantum channels, Physical Review A 99, 032341, (2019). M. Thornton, A. Sakovich, A. Mikhalychev, J. D. Ferrer, P. de la Hoz, N. Korolkova and D. Mogilevtsev: Coherent diffusive photon gun for generating nonclassical states, Physical Review Applied 12, 064051, (2019). S. Richter, M. Thornton, I. Khan, H. Scott, K. Jaksch, U. Vogl, B. Stiller, G. Leuchs, C. Marquardt and N. Korolkova: Agile quantum communication: signatures and secrets, arXiv:2001.10089 [quant-ph]. v CONFERENCEPRESENTATIONS I have had the privilege to present at the following conferences: 1. QCrypt 2016, Washington DC, USA. Poster presentation: “Con- tinuous variable quantum digital signatures.” 2. 6th International Conference on New Frontiers in Physics (ICNFP 2017), Kolymbari, Crete. Oral presentation: “Gigahertz quantum signatures compatible with telecommunication technologies.” 3. QCrypt 2017, Cambridge, UK. Poster presentation: “Gigahertz quantum signatures compatible with telecommunication tech- nologies.” 4. -
Fluctuations, Entropic Quantifiers and Classical-Quantum Transition
Entropy 2014, 16, 1178-1190; doi:10.3390/e16031178 OPEN ACCESS entropy ISSN 1099-4300 www.mdpi.com/journal/entropy Article Fluctuations, Entropic Quantifiers and Classical-Quantum Transition Flavia Pennini 1;2;* and Angelo Plastino 1 1 Instituto de F´ısica La Plata–CCT-CONICET, Universidad Nacional de La Plata, C.C. 727, 1900, La Plata, Argentina; E-Mail: plastino@fisica.unlp.edu.ar 2 Departamento de F´ısica, Universidad Catolica´ del Norte, Av. Angamos 0610, Antofagasta, Chile * Author to whom correspondence should be addressed; E-Mail: [email protected]; Tel./Fax: +54-221-4236335 . Received: 23 December 2013; in revised form: 18 February 2014 / Accepted: 19 February 2014 / Published: 25 February 2014 Abstract: We show that a special entropic quantifier, called the statistical complexity, becomes maximal at the transition between super-Poisson and sub-Poisson regimes. This acquires important connotations given the fact that these regimes are usually associated with, respectively, classical and quantum processes. Keywords: order; semiclassical methods; quantum statistical mechanics; Husimi distributions 1. Introduction This work deals with aspects of the transition between classical and quantum regimes, a subject of much current interest. This is done in terms of an entropic quantifier called the statistical complexity and the methodology employed is the examination of energy fluctuations, that are shown to play a pivotal role in the concomitant considerations. Indeed, our main quantifier (a complexity measure) becomes expressed solely in fluctuations’ terms. We begin by introducing below the main ingredients of our developments. Entropy 2014, 16 1179 1.1. Statistical Complexity A statistical complexity measure (SCM) is a functional that characterizes any given probability distribution P . -
Nonclassical States of Light with a Smooth $P$ Function
PHYSICAL REVIEW A 97, 023832 (2018) Nonclassical states of light with a smooth P function François Damanet,1,2 Jonas Kübler,3 John Martin,2 and Daniel Braun3 1Department of Physics and SUPA, University of Strathclyde, Glasgow G4 0NG, United Kingdom 2Institut de Physique Nucléaire, Atomique et de Spectroscopie, CESAM, Université de Liège, Bâtiment B15, B-4000 Liège, Belgium 3Institut für theoretische Physik, Universität Tübingen, 72076 Tübingen, Germany (Received 12 December 2017; published 21 February 2018) There is a common understanding in quantum optics that nonclassical states of light are states that do not have a positive semidefinite and sufficiently regular Glauber-Sudarshan P function. Almost all known nonclassical states have P functions that are highly irregular, which makes working with them difficult and direct experimental reconstruction impossible. Here we introduce classes of nonclassical states with regular, non-positive-definite P functions. They are constructed by “puncturing” regular smooth positive P functions with negative Dirac-δ peaks or other sufficiently narrow smooth negative functions. We determine the parameter ranges for which such punctures are possible without losing the positivity of the state, the regimes yielding antibunching of light, and the expressions of the Wigner functions for all investigated punctured states. Finally, we propose some possible experimental realizations of such states. DOI: 10.1103/PhysRevA.97.023832 I. INTRODUCTION by Sudarshan [17] that in fact all possible quantum states ρ of the light field (quantum or classical) can be represented Creating and characterizing nonclassical states of light by (1). This is possible due to the fact that coherent states are are two tasks of substantial interest in a wide range of overcomplete. -
Resource Theories of Nonclassical Light
quantum reports Review Resource Theories of Nonclassical Light Kok Chuan Tan * and Hyunseok Jeong * Department of Physics and Astronomy, Seoul National University, Seoul 08826, Korea * Correspondence: [email protected] (K.C.T.); [email protected] (H.J.) Received: 12 September 2019; Accepted: 24 September 2019; Published: 26 September 2019 Abstract: In this focused review we survey recent progress in the development of resource theories of nonclassical light. We introduce the resource theoretical approach, in particular how it pertains to bosonic/light fields, and discuss several different formulations of resource theories of nonclassical light. Keywords: quantum optics; nonclassicality; quantum resource theories; non-Gaussianity 1. Introduction In quantum optics, there is general agreement that the most classical states of light are the coherent states [1–4]. In classical physics, a system can be completely described by a single point in phase space. In quantum mechanics, this is not possible due to the uncertainty principle [5,6]. For this reason, the phase space description of quantum systems must necessarily be via some distribution, rather than a point, that strictly obeys the uncertainty principle. In this sense the coherent state, being a minimum uncertainty state with no preferred axial direction in phase space, is arguably the closest quantum mechanical equivalent to a classical single point description of a physical system. However, one has to bear in mind that coherent states still fundamentally represent a quantum mechanical system. For this reason, despite being the most classical among the quantum states, quantum mechanical properties may still be pried from it. This is demonstrated by their strict observance of the uncertainty principle, as well as by their continued usefulness in purely quantum protocols such as quantum key distribution [7]. -
Lecture 2 5.Pdf
Lecture 5. Instruments of quantum optics. Quantum states. Glauber’s correlation functions. Wigner function and homodyne detection. 1. Quantum states. In the last lecture, we obtained the quantum states generated through quadratic and cubic nonlinear interactions, for the cases of low and high strength. But let us discuss now what other states of light can exist in quantum optics. Operators and states. In quantum mechanics, states are considered as eigenstates (eigenvectors) of various operators. For instance, we already spoke of the photon-number operator Nˆ aˆ aˆ . Clearly, it is a Hermitian operator, Nˆ Nˆ . Any Hermitian operator has real eigenvalues (real spectrum of eigenvalues), forming a complete orthonormal set, and it corresponds to a real (measurable) observable. Accordingly, Nˆ has real eigenvalues and eigenstates: Nˆ N N N . (1) Its eigenstates are photon-number states, or Fock states N . One can show rigorously that N is a natural number,N 0,1,2 ,... For these states, the number of photons is fixed. At the last lecture, we showed that parametric down-conversion and four-wave mixing, at low gain, lead to the generation of such states, although not alone (in superposition with other states). Photon creation and annihilation operators act on Fock states as a N N 1 N 1 , a N N N 1 (ladder equations). It follows that a Fock state can be written as (a ) N N 0 . (2) N! Note that photon-number states form a complete orthonormal set; accordingly, any other state can be written as an expansion over photon-number states. Coherent states are eigenstates of photon creation and annihilation operators, which are non- Hermitian, a a . -
Fundamentals of Quantum Optics
Fundamentals of Quantum Optics Marco Ornigotti Lukas Maczewsky Alexander Szameit Summer Semester 2016 Friedrich-Schiller Universität - Jena June 19, 2016 Contents 1 HAMILTONIAN FORMULATION OF THE ELECTROMAGNETIC FIELD 1 1.1 Coulomb Gauge . 2 1.2 Field Energy Density . 3 2 QUANTIZATION OF THE FREE ELECTROMAGNETIC FIELD 7 3 QUANTUM STATES OF THE ELECTROMAGNETIC FIELD 10 3.1 Number States . 10 3.1.1 Properties of Fock States . 10 3.2 Coherent states . 12 3.2.1 Expectation Value of the electric Field over Coherent States . 13 3.2.2 Action of the Number Operator on Coherent States . 14 3.2.3 Photon Probability . 15 3.2.4 Summary . 15 3.3 Phase Space and Quadrature Operators for the Electromagnetic Field 16 3.3.1 Number states in phase space . 17 3.3.2 Coherent states in phase space . 17 3.4 Displacement Operator . 19 3.5 Squeezed States . 21 4 Interaction of Matter with Quantized Light -I 24 4.1 Electromagnetic Potentials in Presence of Matter . 24 4.2 Introduction of Atoms . 25 4.2.1 Charge Density . 26 4.2.2 Current Density . 26 4.3 Atom-Field Interaction . 27 4.3.1 Electric Part . 28 4.3.2 Magnetic Part . 29 4.3.3 Orders of Magnitude . 29 4.4 The Interaction Hamiltonian . 30 4.5 Second Quantization . 32 4.5.1 Second Quantization of the Atomic Hamiltonian . 32 4.5.2 Dipole Operator . 34 4.5.3 Jaynes-Cummings Model . 35 4.5.4 States Of The Joint System . 37 4.6 Schrödinger, Heisenberg and Interaction Picture . -
Probing Nonclassicality with Matrices of Phase-Space Distributions Martin Bohmann1,2, Elizabeth Agudelo1, and Jan Sperling3
Probing nonclassicality with matrices of phase-space distributions Martin Bohmann1,2, Elizabeth Agudelo1, and Jan Sperling3 1Institute for Quantum Optics and Quantum Information - IQOQI Vienna, Austrian Academy of Sciences, Boltzmanngasse 3, 1090 Vienna, Austria 2QSTAR, INO-CNR, and LENS, Largo Enrico Fermi 2, I-50125 Firenze, Italy 3Integrated Quantum Optics Group, Applied Physics, Paderborn University, 33098 Paderborn, Germany We devise a method to certify nonclassical is closely related to entanglement. Each entangled features via correlations of phase-space distri- state is nonclassical, and single-mode nonclassicality butions by unifying the notions of quasipro- can be converted into two- and multi-mode entangle- babilities and matrices of correlation func- ment [10, 11, 12]. tions. Our approach complements and ex- Consequently, a plethora of techniques to detect tends recent results that were based on Cheby- nonclassical properties have been developed, each shev’s integral inequality [Phys. Rev. Lett. coming with its own operational meanings for appli- 124, 133601 (2020)]. The method developed cations. For example, quantumness criteria which are here correlates arbitrary phase-space functions based on correlation functions and phase-space repre- at arbitrary points in phase space, including sentations have been extensively studied in the con- multimode scenarios and higher-order corre- text of nonclassical light [13, 14]. lations. Furthermore, our approach provides The description of physical systems using the necessary and sufficient nonclassicality crite- phase-space formalism is one of the cornerstones of ria, applies to phase-space functions beyond modern physics [15, 16, 17]. Beginning with ideas in- s-parametrized ones, and is accessible in ex- troduced by Wigner and others [18, 19, 20, 21], the periments. -
Lecture 8. from Nonlinear Optical Effects to Squeezing. 1. Quadrature
Lecture 8. From nonlinear optical effects to squeezing. Squeezing obtained from nonlinear optical effects: PDC and FWM, Kerr effect. Passing from quadrature to twin-beam squeezing and vice versa. Obtaining sub-Poissonian statistics through feedforward technique and through second harmonic generation. The role of loss. 1. Quadrature squeezing. In this lecture we will continue discussing how nonclassical light can be produced through PDC and FWM. Unlike in the previous lecture, now we focus on high-gain effects and bright light. Therefore the criteria for nonclassicality will be different now: squeezing, sub- Poissonian photon statistics, and sub-shot-noise photon-number correlations (noise reduction). Parametric down-conversion. Consider the degenerate Hamiltonian, ˆ 2 H i(a ) h.c. (1) In Lecture 4 we have derived that this Hamiltonian makes the quadratures evolve as qˆ eGqˆ , pˆ eG pˆ , 0 0 (2) G 2t. Through this evolution, an input vacuum state becomes squeezed vacuum, see Fig.2 in Lecture 4. One can describe it by acting on the input vacuum state by the evolution operator, 1 G exp{ dtHˆ } exp{ (a )2 h.c.} Sˆ(G) , i 2 where we introduced the squeezing operator Sˆ(G). Then the squeezed state can be written as Sˆ(G) vac . (3) To observe this squeezing, one should perform homodyne tomography and measure the Wigner function (Lecture 5). The nonclassical feature is then the uncertainty of a quadrature being below the shot noise. A measure of this nonclassicality is quadrature squeezing in dB, defined as 2 p 2G 10log 2 10log[e ]. p0 (We assume here that it is the p quadrature that is squeezed; in the general case it can be any quadrature.) The record quadrature squeezing achieved now is -15 dB [H. -
Polarization Squeezing and Continuous- Variable Polarization Entanglement
Polarization squeezing and continuous- variable polarization entanglement Natalia Korolkova,1 Gerd Leuchs,1 Rodney Loudon,1,2 Timothy C. Ralph3 and Christine Silberhorn1 1Lehrstuhl für Optik, Physikalisches Institut, Friedrich–Alexander–Universität, Staudtstr. 7/B2, D–91058 Erlangen, Germany 2Department of Electronic Systems Engineering, Essex University, Colchester CO4 3SQ, UK 3Department of Physics, University of Queensland, St Lucia, QLD 4072, Australia The Stokes-parameter operators and the associated Poincaré sphere, which describe the quantum-optical polarization properties of light, are defined and their basic properties are re- viewed. The general features of the Stokes operators are illustrated by evaluation of their means and variances for a range of simple polarization states. Some of the examples show polarization squeezing, in which the variances of one or more Stokes parameters are smaller than the coherent-state value. The main object of the paper is the application of these concepts to bright squeezed light. It is shown that a light beam formed by interference of two orthogonally-polarized quadrature-squeezed beams exhibits squeezing in some of the Stokes parameters. Passage of such a primary polarization-squeezed beam through suitable optical components generates a pair of polarization-entangled light beams with the nature of a two- mode squeezed state. The use of pairs of primary polarization-squeezed light beams leads to substantially increased entanglement and to the generation of EPR-entangled light beams. The important advantage of these nonclassical polarization states for quantum communication is the possibility of experimentally determining all of the relevant conjugate variables of both squeezed and entangled fields using only linear optical elements followed by direct detection.