Single Cell Biophysics: Applications in Cardiomyocyte Mechanobiology and Stem Cell Mechanotransduction
Total Page:16
File Type:pdf, Size:1020Kb
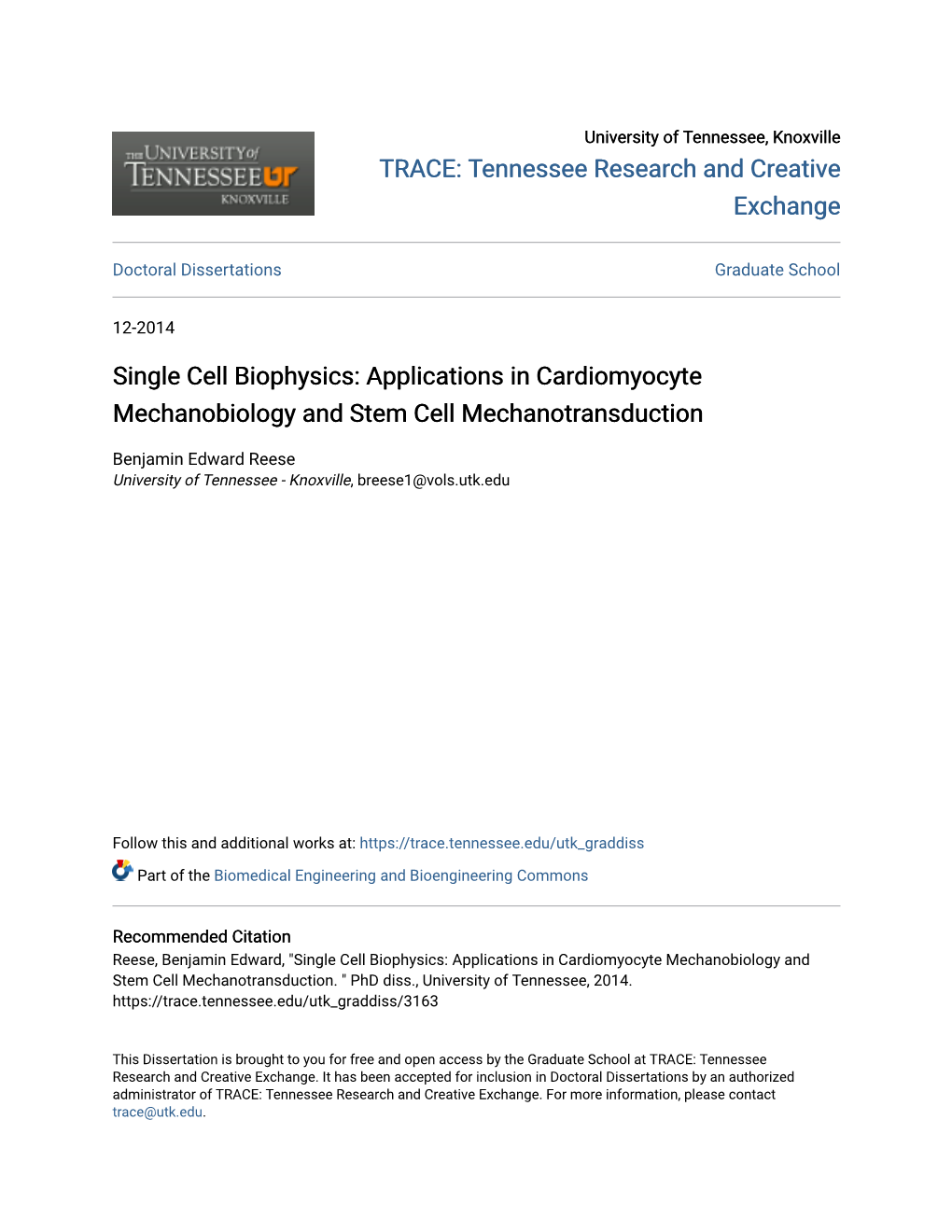
Load more
Recommended publications
-
Melanoma in the Eyes of Mechanobiology
fcell-08-00054 February 11, 2020 Time: 16:43 # 1 REVIEW published: 11 February 2020 doi: 10.3389/fcell.2020.00054 Melanoma in the Eyes of Mechanobiology M. Manuela Brás1,2,3, Manfred Radmacher4*, Susana R. Sousa1,2,5 and Pedro L. Granja1,2,3† 1 Instituto de Investigação e Inovação em Saúde, Universidade do Porto, Porto, Portugal, 2 Instituto de Engenharia Biomédica, Universidade do Porto, Porto, Portugal, 3 Faculdade de Engenharia, Universidade do Porto, Porto, Portugal, 4 Institute for Biophysics, University of Bremen, Bremen, Germany, 5 Instituto Superior de Engenharia do Porto, Instituto Politécnico do Porto, Porto, Portugal Skin is the largest organ of the human body with several important functions that can be impaired by injury, genetic or chronic diseases. Among all skin diseases, melanoma is one of the most severe, which can lead to death, due to metastization. Mechanotransduction has a crucial role for motility, invasion, adhesion and metastization processes, since it deals with the response of cells to physical forces. Signaling Edited by: pathways are important to understand how physical cues produced or mediated Shamik Sen, by the Extracellular Matrix (ECM), affect healthy and tumor cells. During these Indian Institute of Technology Bombay, India processes, several molecules in the nucleus and cytoplasm are activated. Melanocytes, Reviewed by: keratinocytes, fibroblasts and the ECM, play a crucial role in melanoma formation. This Zhizhan Gu, manuscript will address the synergy among melanocytes, keratinocytes, fibroblasts cells Dana-Farber Cancer Institute, United States and the ECM considering their mechanical contribution and relevance in this disease. Takashi Kato, Mechanical properties of melanoma cells can also be influenced by pigmentation, Johns Hopkins University, which can be associated with changes in stiffness. -
Quantitative Methodologies to Dissect Immune Cell Mechanobiology
cells Review Quantitative Methodologies to Dissect Immune Cell Mechanobiology Veronika Pfannenstill 1 , Aurélien Barbotin 1 , Huw Colin-York 1,* and Marco Fritzsche 1,2,* 1 Kennedy Institute for Rheumatology, University of Oxford, Roosevelt Drive, Oxford OX3 7LF, UK; [email protected] (V.P.); [email protected] (A.B.) 2 Rosalind Franklin Institute, Harwell Campus, Didcot OX11 0FA, UK * Correspondence: [email protected] (H.C.-Y.); [email protected] (M.F.) Abstract: Mechanobiology seeks to understand how cells integrate their biomechanics into their function and behavior. Unravelling the mechanisms underlying these mechanobiological processes is particularly important for immune cells in the context of the dynamic and complex tissue microen- vironment. However, it remains largely unknown how cellular mechanical force generation and mechanical properties are regulated and integrated by immune cells, primarily due to a profound lack of technologies with sufficient sensitivity to quantify immune cell mechanics. In this review, we discuss the biological significance of mechanics for immune cells across length and time scales, and highlight several experimental methodologies for quantifying the mechanics of immune cells. Finally, we discuss the importance of quantifying the appropriate mechanical readout to accelerate insights into the mechanobiology of the immune response. Keywords: mechanobiology; biomechanics; force; immune response; quantitative technology Citation: Pfannenstill, V.; Barbotin, A.; Colin-York, H.; Fritzsche, M. Quantitative Methodologies to Dissect 1. Introduction Immune Cell Mechanobiology. Cells The development of novel quantitative technologies and their application to out- 2021, 10, 851. https://doi.org/ standing scientific problems has often paved the way towards ground-breaking biological 10.3390/cells10040851 findings. -
Mechanobiology of Migrating Cells from Basic Science to the Clinic
A COST Action CA15214 (EuroCellNet) Working Group Meeting Mechanobiology of Migrating Cells From Basic Science to the Clinic 1st – 2nd April 2019 Venue Department of Physics Rua Larga, University of Coimbra 2 Coimbra 2019 Monday, 1st April 09.45 - 10.00 WELCOME AND INTRODUCTION Keynote Speaker 10.00 – 10.40 M. Ángela Nieto, Instituto de Neurociencias (CSIC-UMH), Alicante, Spain “Epithelial plasticity in health and disease (the INs and OUTs of the EMT)” ECM and Cell Migration Session Chair: Nuno Saraiva 10.40 – 11.00 Florence Janody, University of Porto, Portugal “Computational modelling and experimental approaches identify a role of ECM stiffening in Src- induces EMT” 11.00 - 11.20 Juan Carlos Rodríguez-Manzaneque, GENYO, Granada, Spain “Relevance of ECM proteolytic remodelling for cell invasion and migration” 11.20 - 11.40 Rui Travasso, University of Coimbra, Portugal “Mathematical modelling of migrating cells and angiogenesis” 11.40 - 12.00 María José Oliveira, University of Porto, Portugal “Decellularized human colorectal cancer matrices as a tumor microenvironment biomimetic model” 12.00 – 14.00 Lunch Justiça e Paz, Couraça de Lisboa, 30 Keynote Speaker 14.00 - 14.40 Lino Ferreira, University of Coimbra, Portugal “Mechanical forces in vascular cell maturation and disease” 4 Coimbra 2019 Mechanotransduction and Cytoskeleton Session Chair: Ana Fernandes 14.40 - 15.00 Mirjana Liovic, Medical Center for Molecular Biology, Institute for Biochemistry, Faculty of Medicine, University of Ljubljana, Ljubljana, Slovenia “Cytoskeletal mutations -
Integrated Micro/Nanoengineered Functional Biomaterials for Cell Mechanics and Mechanobiology: REVIEW a Materials Perspective
www.advmat.de www.MaterialsViews.com Integrated Micro/Nanoengineered Functional Biomaterials for Cell Mechanics and Mechanobiology: REVIEW A Materials Perspective Yue Shao and Jianping Fu * which actively signals to cells to regulate The rapid development of micro/nanoengineered functional biomaterials in their fate and function. The microenviron- the last two decades has empowered materials scientists and bioengineers mental factors, including cell-cell inter- to precisely control different aspects of the in vitro cell microenvironment. actions, soluble factors such as oxygen tension and growth factors, and adhesive Following a philosophy of reductionism, many studies using synthetic and biophysical interactions between functional biomaterials have revealed instructive roles of individual extra- cells and extracellular matrix (ECM), are cellular biophysical and biochemical cues in regulating cellular behaviors. all important for regulation of cellular Development of integrated micro/nanoengineered functional biomaterials behaviors. Cells and the surrounding to study complex and emergent biological phenomena has also thrived microenvironment can also dynamically infl uence each other during normal devel- rapidly in recent years, revealing adaptive and integrated cellular behaviors opment, tissue homeostasis and repair, closely relevant to human physiological and pathological conditions. Working and progression of diseases through their at the interface between materials science and engineering, biology, and reciprocal biochemical and biophysical medicine, we are now at the beginning of a great exploration using micro/ interactions. [ 1–6 ] Thus, a detailed appre- nanoengineered functional biomaterials for both fundamental biology study hension and understanding of cell-micro- and clinical and biomedical applications such as regenerative medicine and environment interactions is critical for both advancing basic biology knowledge drug screening. -
Modeling the Mechanobiology of Cancer Cell Migration Using 3D Biomimetic Hydrogels
gels Review Modeling the Mechanobiology of Cancer Cell Migration Using 3D Biomimetic Hydrogels Xabier Morales †, Iván Cortés-Domínguez † and Carlos Ortiz-de-Solorzano * IDISNA, Ciberonc and Solid Tumors and Biomarkers Program, Center for Applied Medical Research, University of Navarra, 31008 Pamplona, Spain; [email protected] (X.M.); [email protected] (I.C.-D.) * Correspondence: [email protected] † These authors contributed equally to this work. Abstract: Understanding how cancer cells migrate, and how this migration is affected by the me- chanical and chemical composition of the extracellular matrix (ECM) is critical to investigate and possibly interfere with the metastatic process, which is responsible for most cancer-related deaths. In this article we review the state of the art about the use of hydrogel-based three-dimensional (3D) scaffolds as artificial platforms to model the mechanobiology of cancer cell migration. We start by briefly reviewing the concept and composition of the extracellular matrix (ECM) and the materials commonly used to recreate the cancerous ECM. Then we summarize the most relevant knowledge about the mechanobiology of cancer cell migration that has been obtained using 3D hydrogel scaf- folds, and relate those discoveries to what has been observed in the clinical management of solid tumors. Finally, we review some recent methodological developments, specifically the use of novel bioprinting techniques and microfluidics to create realistic hydrogel-based models of the cancer ECM, and some of their applications in the context of the study of cancer cell migration. Keywords: hydrogel; collagen; Matrigel; extracellular matrix; mechanobiology; amoeboid-mesenchymal transition; cancer; cell migration; microfluidic devices; bioprinting Citation: Morales, X.; Cortés-Domínguez, I.; Ortiz-de-Solorzano, C. -
A Novel Role for Cadherin-11
Mechanobiology of Cardiac Disease and Fibrosis: a Novel Role for Cadherin-11 By Alison Koelle Schroer Dissertation Submitted to the Faculty of the Graduate School of Vanderbilt University in partial fulfillment of the requirements for the degree of DOCTOR OF PHILOSOPHY in Biomedical Engineering December, 2016 Nashville, Tennessee Approved: W. David Merryman, Ph.D. John Wikswo, Ph.D. Michael Miga, Ph.D. Jeffrey Davidson, Ph.D. Antonis Hatzopoulos, Ph.D. Copyright © 2016 by Alison K Schroer All Rights Reserved ii ACKNOWLEDGEMENTS The heart has its reasons, of which reason knows nothing - Pascal I must acknowledge my coauthors on the manuscripts which have been adapted and included in this dissertation. First and foremost, my advisor Dave Merryman. Also Larisa Rhyzhova, Cyndi Clark, Hind Lal, Qinkun Zhang, Tom Force, John Wikswo, Veniamin Sidorov, Matthew Shotwell, Annabelle Manalo, and David Bader. I would also acknowledge Josh Bender and Claire Lafferty, undergraduate research assistants who assisted in the collection of some of the data included in this work. I would also like to acknowledge Meghan Bowler, Mark Vander Roest, Caleb Snider, Allison Price, and Jeffrey Davidson for their editorial comments on the work included in this dissertation. I would like to acknowledge my funding sources, especially the NSF and the AHA. Also, the ever wonderful Merryman Lab, members both past and present, who have been my comrades and friends throughout the last five years. Finally, I must acknowledge my family, my friends, and my God, without whom I never could have done all this. Keep your heart with all vigilance, for from it flow the springs of life. -
Cellular Mechanobiology of Glioblastoma Multiforme
Cellular Mechanobiology of Glioblastoma Multiforme by Theresa Ann Ulrich A dissertation submitted in partial satisfaction of the requirements of the degree of Joint Doctor of Philosophy with University of California, San Francisco in Bioengineering in the Graduate Division of the University of California, Berkeley Committee in charge: Professor Sanjay Kumar, Chair Professor Mohammad Mofrad Professor Tejal Desai Professor Andrew Wurmser Spring 2011 Cellular Mechanobiology of Glioblastoma Multiforme © 2011 By Theresa Ann Ulrich Abstract Cellular Mechanobiology of Glioblastoma Multiforme by Theresa Ann Ulrich Joint Doctor of Philosophy in Bioengineering with University of California, San Francisco University of California, Berkeley Professor Sanjay Kumar, Chair The rapid progression of high-grade brain tumors is related to diffuse infiltration of single tumor cells into the surrounding brain parenchyma, a process that involves aberrant interactions between tumor cells and the extracellular matrix (ECM). Tremendous effort has been devoted to elucidating the genetic and biochemical underpinnings of these tumors; however, poor translation of candidate therapies from animal models to human patients has only increased the sense of urgency for the development of new approaches in both the laboratory and the clinic. Indeed, despite decades of extensive clinical and biological research, the life expectancy of patients with grade IV glioblastoma multiforme (GBM) brain tumors is still approximately one year at diagnosis. The work presented in this dissertation has approached this problem from a biophysical perspective, demonstrating that biomechanical cues from the ECM serve as regulators of key GBM tumor cell properties relevant to invasion in both two-dimensional (2D) and three-dimensional (3D) culture models. We first investigated the role of ECM rigidity in regulating the structure, migration, and proliferation of a panel of glioma cell lines on 2D fibronectin-coated polymeric ECM substrates of defined mechanical rigidity. -
Wang (2006) Mechanobiology of Tendon.Pdf
ARTICLE IN PRESS Journal of Biomechanics 39 (2006) 1563–1582 www.elsevier.com/locate/jbiomech www.JBiomech.com Review Mechanobiology of tendon James H.-C. Wangà MechanoBiology Laboratory, Departments of Orthopaedic Surgery, Bioengineering and Mechanical Engineering, University of Pittsburgh, 210 Lothrop St., BST, E1647, Pittsburgh, PA 15213, USA Accepted 11 May 2005 Abstract Tendons are able to respond to mechanical forces by altering their structure, composition, and mechanical properties—a process called tissue mechanical adaptation. The fact that mechanical adaptation is effected by cells in tendons is clearly understood; however, how cells sense mechanical forces and convert them into biochemical signals that ultimately lead to tendon adaptive physiological or pathological changes is not well understood. Mechanobiology is an interdisciplinary study that can enhance our understanding of mechanotransduction mechanisms at the tissue, cellular, and molecular levels. The purpose of this article is to provide an overview of tendon mechanobiology. The discussion begins with the mechanical forces acting on tendons in vivo, tendon structure and composition, and its mechanical properties. Then the tendon’s response to exercise, disuse, and overuse are presented, followed by a discussion of tendon healing and the role of mechanical loading and fibroblast contraction in tissue healing. Next, mechanobiological responses of tendon fibroblasts to repetitive mechanical loading conditions are presented, and major cellular mechanotransduction mechanisms are briefly reviewed. Finally, future research directions in tendon mechanobiology research are discussed. r 2005 Elsevier Ltd. All rights reserved. Keywords: Tendon; Mechanobiology; Mechanical adaptation; Tendon fibroblasts; Mechanotransduction Contents 1. Introduction . 1564 2. Tendon forces in vivo . 1564 3. Tendon structure, composition, and mechanical properties . -
Mechanobiology and Mechanics in Cardiovascular Disease
obiolog Bae, J Microbiol Pathol 2018, 2:1 icr y & M P f a o t l h a o n l o r g u y o J Journal of Microbiology and Pathology EditorialResearch Article OpenOpen Access Access Mechanobiology and Mechanics in Cardiovascular Disease Yongho Bae* Department of Pathology and Anatomical Sciences, Jacobs School of Medicine and Biomedical Sciences, University at Buffalo, State University of New York, USA Cardiovascular disease is the main cause of death globally. Arterial controls vascular smooth muscle cell function and to determine how stiffening significantly contributes to the progression of cardiovascular pathological arterial stiffness disrupts that control which contributes disease [1-3] including atherosclerosis, coronary heart disease, to the progression of cardiovascular disease. These research findings hypertension and stroke. The molecular mechanisms governing will be significantly relevant for clinical revascularization procedures arterial stiffening and the phenotypic changes in vascular smooth (angioplasty and stenting), where the risk of restenosis attributable to muscle cells associated with the stiffening process are critical areas in neointimal formation may be mitigated by targeting certain mechano cardiovascular biology, mechanics and pathology. Evidence suggests sensitive proteins to maintain lumen diameter, vessel function, and that arterial stiffness can drive aberrant vascular smooth muscle cell physiological arterial stiffness. Thus, current and future work by dedifferentiation, migration, and proliferation within the vessel wall researchers may lead to new therapies for treating cardiovascular [4-9]. Yet, the underlying mechanisms regulating arterial stiffening and disease. the molecular changes within vascular smooth muscle cells associated References with the stiffening process remain unclear. 1. Mitchell GF, Hwang SJ, Vasan RS, Larson MG, Pencina MJ, et al. -
Cell Mechanics and Mechanobiology
CELL MECHANICS AND MECHANOBIOLOGY Hans Van Oosterwyck Biomechanics section, Department of Mechanical Engineering, KU Leuven, Belgium Liesbet Geris Biomechanics Research Unit, U.Liège, Belgium José Manuel García Aznar Multiscale in Mechanical and Biological Engineering (M2BE), Aragón Institute of Engineering Research (I3A), Universidad de Zaragoza, Spain Key Words: mechanobiology, cell mechanics, mechanotransduction, cytoskeleton, computational modelling. Contents 1. Introduction 2. Cell mechanics 2.1. Overview 2.2. Experimental techniques to measure cell mechanical properties 2.3. Computational modelling of cell mechanical properties 3. Cell mechanobiology 3.1. Overview 3.2. Mechanotransduction 3.3. Computational modelling of cell mechanobiology 4. Conclusions Acknowledgements Glossary Bibliography Biographical Sketches Summary Mechanical signals are important regulators of cell behaviour. Key to understanding their role is the fact that cells are able to sense and respond to mechanical signals. In order to unravel the interplay between mechanics and biology one needs to embrace experimental and computational methods, stemming from engineering as well as biological disciplines, and integrate them into an interdisciplinary research field called mechanobiology. In this chapter we will first describe the structural and mechanical properties of a cell and its components, as these properties will have important consequences for the way mechanical signals are converted into a biochemical response. Experimental techniques to measure and computational models to capture these properties will be highlighted. Once we have addressed some key aspects of cell mechanics, we will continue by describing some key mechanisms of how mechanical signals can modulate cell behaviour. Again, insights from experimental as well as computational studies will be reviewed. Given the broadness of the field, we will either focus on generic mechanisms, or limit ourselves to a few examples and case studies. -
Mechanobiology of Myofibroblast Adhesion in Fibrotic Cardiac Disease Alison K
© 2015. Published by The Company of Biologists Ltd | Journal of Cell Science (2015) 128, 1865-1875 doi:10.1242/jcs.162891 COMMENTARY ARTICLE SERIES: CELL BIOLOGY AND DISEASE Mechanobiology of myofibroblast adhesion in fibrotic cardiac disease Alison K. Schroer1 and W. David Merryman1,* ABSTRACT behavior and phenotype of cardiac cells, which contributes to Fibrotic cardiac disease, a leading cause of death worldwide, manifests maladaptive tissue remodeling (Cuniberti et al., 2006; Merryman as substantial loss of function following maladaptive tissue remodeling. et al., 2006). Therefore, determining how various cardiac cells Fibrosis can affect both the heart valves and the myocardium and respond to changing mechanical environments will aid our is characterized by the activation of fibroblasts and accumulation of understanding of the development of heart disease and potentially extracellular matrix. Valvular interstitial cells and cardiac fibroblasts, the uncover new targets for future therapy (Fig. 1A). cell types responsible for maintenance of cardiac extracellular matrix, Valvular interstitial cells (VICs) and cardiac fibroblasts (CFs) are are sensitive to changing mechanical environments, and their ability to primarily responsible for maintaining the ECM, and are sensitive to sense and respond to mechanical forces determines both normal mechanical forces in addition to chemical cues. Mechanically induced development and the progression of disease. Recent studies have signaling promotes myofibroblast (MyoFB) differentiation of VICs uncovered specific adhesion proteins and mechano-sensitive signaling and CFs, resulting in cells that exhibit increased contractility and pathways that contribute to the progression of fibrosis. Integrins form increased secretion of growth factors and ECM proteins (Fig. 1B) adhesions with the extracellular matrix, and respond to changes in (Tomasek et al., 2002). -
Physical Therapists As a Force in Mechanotherapy and Musculoskeletal Regenerative Rehabilitation W.R
Regenerative Rehabilitation Special Issue Understanding Mechanobiology: Physical Therapists as a Force in Mechanotherapy and Musculoskeletal Regenerative Rehabilitation W.R. Thompson, PT, DPT, PhD, William R. Thompson, Alexander Scott, M. Terry Loghmani, Samuel R. Ward, Department of Physical Therapy, Stuart J. Warden School of Health and Rehabilita- tion Sciences, Indiana University, Indianapolis, Indiana. Achieving functional restoration of diseased or injured tissues is the ultimate goal of both A. Scott, PT, PhD, Department of regenerative medicine approaches and physical therapy interventions. Proper integration and Physical Therapy, Faculty of Med- healing of the surrogate cells, tissues, or organs introduced using regenerative medicine icine, University of British Colum- techniques are often dependent on the co-introduction of therapeutic physical stimuli. Thus, bia, Vancouver, British Columbia, regenerative rehabilitation represents a collaborative approach whereby rehabilitation special- Canada. ists, basic scientists, physicians, and surgeons work closely to enhance tissue restoration by M.T. Loghmani, PT, PhD, Depart- creating tailored rehabilitation treatments. One of the primary treatment regimens that phys- ment of Physical Therapy, School ical therapists use to promote tissue healing is the introduction of mechanical forces, or of Health and Rehabilitation Sci- mechanotherapies. These mechanotherapies in regenerative rehabilitation activate specific ences, Indiana University. biological responses in musculoskeletal tissues