General Disclaimer One Or More of the Following Statements May Affect
Total Page:16
File Type:pdf, Size:1020Kb
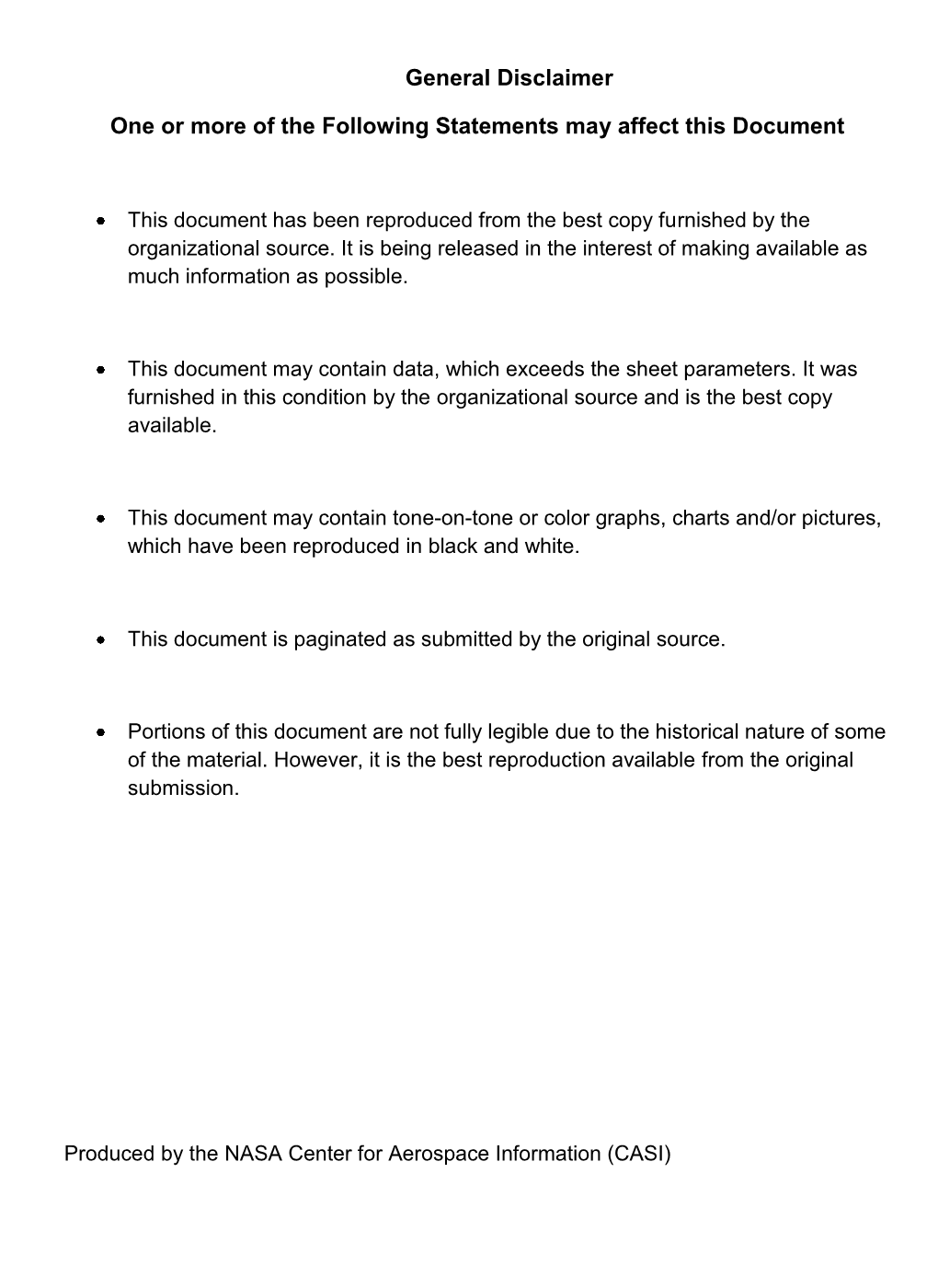
Load more
Recommended publications
-
Cosmicflows-3: Cosmography of the Local Void
Draft version May 22, 2019 Preprint typeset using LATEX style AASTeX6 v. 1.0 COSMICFLOWS-3: COSMOGRAPHY OF THE LOCAL VOID R. Brent Tully, Institute for Astronomy, University of Hawaii, 2680 Woodlawn Drive, Honolulu, HI 96822, USA Daniel Pomarede` Institut de Recherche sur les Lois Fondamentales de l'Univers, CEA, Universite' Paris-Saclay, 91191 Gif-sur-Yvette, France Romain Graziani University of Lyon, UCB Lyon 1, CNRS/IN2P3, IPN Lyon, France Hel´ ene` M. Courtois University of Lyon, UCB Lyon 1, CNRS/IN2P3, IPN Lyon, France Yehuda Hoffman Racah Institute of Physics, Hebrew University, Jerusalem, 91904 Israel Edward J. Shaya University of Maryland, Astronomy Department, College Park, MD 20743, USA ABSTRACT Cosmicflows-3 distances and inferred peculiar velocities of galaxies have permitted the reconstruction of the structure of over and under densities within the volume extending to 0:05c. This study focuses on the under dense regions, particularly the Local Void that lies largely in the zone of obscuration and consequently has received limited attention. Major over dense structures that bound the Local Void are the Perseus-Pisces and Norma-Pavo-Indus filaments sepa- rated by 8,500 km s−1. The void network of the universe is interconnected and void passages are found from the Local Void to the adjacent very large Hercules and Sculptor voids. Minor filaments course through voids. A particularly interesting example connects the Virgo and Perseus clusters, with several substantial galaxies found along the chain in the depths of the Local Void. The Local Void has a substantial dynamical effect, causing a deviant motion of the Local Group of 200 − 250 km s−1. -
Arxiv:0705.4139V2 [Astro-Ph] 14 Dec 2007 the Bulk Motion of the Local Sheet Away from the Local Void
Our Peculiar Motion Away from the Local Void R. Brent Tully, Institute for Astronomy, University of Hawaii, 2680 Woodlawn Drive, Honolulu, HI 96822 and Edward J. Shaya University of Maryland, Astronomy Department, College Park, MD 20743 and Igor D. Karachentsev. Special Astrophysical Observatory, Nizhnij Arkhyz, Karachaevo-Cherkessia, Russia and H´el`eneM. Courtois, Dale D. Kocevski, and Luca Rizzi Institute for Astronomy, University of Hawaii, 2680 Woodlawn Drive, Honolulu, HI 96822 and Alan Peel University of Maryland, Astronomy Department, College Park, MD 20743 ABSTRACT The peculiar velocity of the Local Group of galaxies manifested in the Cosmic Microwave Background dipole is found to decompose into three dominant components. The three compo- nents are clearly separated because they arise on distinct spatial scales and are fortuitously almost orthogonal in their influences. The nearest, which is distinguished by a velocity discontinuity at ∼ 7 Mpc, arises from the evacuation of the Local Void. We lie in the Local Sheet that bounds the void. Random motions within the Local Sheet are small and we advocate a reference frame with respect to the Local Sheet in preference to the Local Group. Our Galaxy participates in arXiv:0705.4139v2 [astro-ph] 14 Dec 2007 the bulk motion of the Local Sheet away from the Local Void. The component of our motion on an intermediate scale is attributed to the Virgo Cluster and its surroundings, 17 Mpc away. The third and largest component is an attraction on scales larger than 3000 km s−1 and centered near the direction of the Centaurus Cluster. The amplitudes of the three components are 259, 185, and 455 km s−1, respectively, adding collectively to 631 km s−1 in the reference frame of the Local Sheet. -
Download This Article in PDF Format
A&A 577, A144 (2015) Astronomy DOI: 10.1051/0004-6361/201526054 & c ESO 2015 Astrophysics A graph of dark energy significance on different spatial and mass scales (Research Note) P. Teerikorpi1, P. Heinämäki1,P.Nurmi1,A.D.Chernin2, M. Einasto3,M.Valtonen1, and G. Byrd4 1 Tuorla Observatory, Department of Physics and Astronomy, University of Turku, 21500 Piikkiö, Finland e-mail: [email protected] 2 Sternberg Astronomical Institute, Moscow University, 119899 Moscow, Russia 3 Tartu Observatory, 61602 Tõravere, Estonia 4 University of Alabama, Tuscaloosa, AL 35487-0324, USA Received 9 March 2015 / Accepted 21 March 2015 ABSTRACT Context. The current cosmological paradigm sees the formation and evolution of the cosmic large-scale structure as governed by the gravitational attraction of dark matter (DM) and the repulsion of dark energy (DE). Aims. We characterize the relative importance of uniform and constant dark energy, as given by the Λ term in the standard ΛCDM cos- mology, in galaxy systems of different scales from groups to superclusters. Methods. An instructive “Λ significance graph” is introduced where the matter-DE density ratio ρM/ρΛ for different galaxy systems is plotted against the radius R. This presents gravitation- and DE-dominated regions and directly shows the zero velocity radius, the zero-gravity radius, and the Einstein-Straus radius for any fixed value of mass. Results. Example galaxy groups and clusters from the local universe illustrate the use of the Λ significance graph. These are generally located deep in the gravity-dominated region ρM/ρΛ > 2, and are virialized. Extended clusters and the main bodies of superclusters can reach down near the borderline between gravity-dominated and DE-dominated regions ρM/ρΛ = 2. -
Arxiv:1306.0091V4 [Astro-Ph.CO] 13 Jun 2013
Draft version June 14, 2013 A Preprint typeset using LTEX style emulateapj v. 5/2/11 COSMOGRAPHY OF THE LOCAL UNIVERSE Hel´ ene` M. Courtois1,2 1University of Lyon; UCB Lyon 1/CNRS/IN2P3; IPN Lyon, France and 2Institute for Astronomy (IFA), University of Hawaii, 2680 Woodlawn Drive, HI 96822, USA Daniel Pomarede` 3 3CEA/IRFU, Saclay, 91191 Gif-sur-Yvette, France R. Brent Tully2 1Institute for Astronomy (IFA), University of Hawaii, 2680 Woodlawn Drive, HI 96822, USA Yehuda Hoffman4 4Racah Institute of Physics, Hebrew University, Jerusalem 91904, Israel and Denis Courtois5 Lyc´ee international, 38300 Nivolas-vermelle, France Draft version June 14, 2013 ABSTRACT The large scale structure of the universe is a complex web of clusters, filaments, and voids. Its properties are informed by galaxy redshift surveys and measurements of peculiar velocities. Wiener Filter reconstructions recover three-dimensional velocity and total density fields. The richness of the elements of our neighborhood are revealed with sophisticated visualization tools. A key component of this paper is an accompanying movie. The ability to translate and zoom helps the viewer fol- low structures in three dimensions and grasp the relationships between features on different scales while retaining a sense of orientation. The ability to dissolve between scenes provides a technique for comparing different information, for example, the observed distribution of galaxies, smoothed repre- sentations of the distribution accounting for selection effects, observed peculiar velocities, smoothed and modeled representations of those velocities, and inferred underlying density fields. The agreement between the large scale structure seen in redshift surveys and that inferred from reconstructions based on the radial peculiar velocities of galaxies strongly supports the standard model of cosmology where structure forms from gravitational instabilities and galaxies form at the bottom of potential wells. -
Spin Alignment in Analogues of the Local Sheet George J
The Zeldovich Universe: Genesis and Growth of the Cosmic Web Proceedings IAU Symposium No. 308, 2014 c International Astronomical Union 2016 R. van de Weygaert, S. Shandarin, E. Saar & J. Einasto, eds. doi:10.1017/S1743921316010334 Spin Alignment in Analogues of The Local Sheet George J. Conidis Department of Physics and Astronomy, York University, Toronto, Ontario, M3J 1P3, Canada email: [email protected] Abstract. Tidal torque theory and simulations of large scale structure predict spin vectors of massive galaxies should be coplanar with sheets in the cosmic web. Recently demonstrated, the giants (Ks -22.5 mag) in the Local Volume beyond the Local Sheet have spin vectors directed close to the plane of the Local Supercluster, supporting the predictions of Tidal Torque Theory. However, the giants in the Local Sheet encircling the Local Group display a distinctly different arrangement, suggesting that the mass asymmetry of the Local Group or its progenitor torqued them from their primordial spin directions. To investigate the origin of the spin alignment of giants locally, analogues of the Local Sheet were identified in the SDSS DR9. Similar to the Local Sheet, analogues have an interacting pair of disk galaxies isolated from the remaining sheet members. Modified sheets in which there is no interacting pair of disk galaxies were identified as a control sample. Galaxies in face-on control sheets do not display axis ratios predominantly weighted toward low values, contrary to the expectation of tidal torque theory. For face-on and edge-on sheets, the distribution of axis ratios for galaxies in analogues is distinct from that in controls with a confidence of 97.6 % & 96.9%, respectively. -
Cosmography of the Local Universe: Narration of the Movie Authors: H.M. Courtois, D. Pomarede, R.B. Tully, Y. Hoffman, D. Courto
Cosmography of the Local Universe: Narration of The Movie Authors: H.M. Courtois, D. Pomarede, R.B. Tully, Y. Hoffman, D. Courtois Article : http://arxiv.org/abs/1306.0091 , In Press in Astronomical Journal, June 2013 Movie that can be viewed and downloaded at : http://irfu.cea.fr/cosmography or http://vimeo.com/pomarede/cosmography Fig 1 We start our adventure with a view of the projection of all galaxies within 8000 km/s in the V8k redshift catalog. The projection is in supergalactic coordinates with the plane of the Milky Way slanting at a steep angle across the center and wrapping around the edges. The nearest galaxies are in dark blue and concentrate : around the Virgo Cluster on the supergalactic equator to the right of center, or around the Fornax Cluster in the lower left quadrant. One of the two most prominent filaments is the Pavo-Indus structure in cyan and yellow that can be followed from near the Virgo Cluster, across the zone of obscuration, rising increasingly above the equator toward the left. The other most prominent structure is the Perseus-Pisces filament in yellow and red, rising at a sharp angle to the extreme left. Fig 2 ======================== Where do these galaxies lie in three dimensions? That’s seen as we transfer galaxies from the two-dimensional projection to a three-dimensional cube. The galaxies end up at their positions in redshift space. Kinematic stretching in clusters, has been truncated. Positions of galaxies within 3000 km/s have been modified in accordance with a model of galaxy streaming around the Virgo Cluster. -
Laniakea Supercluster
WISE @Laniakea 5 Supercluster and beyond with WISE Don Neill, Frank Masci - Caltech Cosmicflows-2 Mark Seibert - Carnegie Obs. Cosmic Flows Program ! • Measure distances d" • Peculiar velocities: Vpec = Vobs - H0d" • Infer 3D velocities and density field" • Project to initial conditions" • Simulate evolution to present conditions Gottloeber, Hoffman, Klypin, Yepes (CLUES collaboration)" Sorce, Kitaura the local volume 1. tiny peculiar velocities within Local Sheet" 2. discontinuity in peculiar velocities passing to adjacent structures" 3. 185 km/s motion toward Virgo Cluster" 4. 260 km/s motion away from Local Void" the local volume Latest data, Local Group frame After removal of local motion Cosmicflows-1: 1797 distances within 3300 km/s " (catalog in EDD) Tully et al. 2008, ApJ, 676, 184" Contributions to Cosmicflows-2 297 TRGB: Tip of the Red Giant Branch" 133 TRGB Literature" 31 RR Lyr, Horiz Br, Eclip Bin, Maser" 1209" 60 Cepheid Period-Luminosity" 382 SBF: Surface Brightness Fluctuation" 306 SNIa: Type Ia Supernova" 1508 FP: Fundamental Plane" 5998 TF: Luminosity-Linewidth " " 8315 distance measures within 30,000 km/s" BT, Courtois, Dolphin, Fisher, Heraudeau, Jacobs, Karachentsev, Makarov, Makarova, Mitronova, Rizzi, Shaya, Sorce, Wu" 2013, AJ, 146, 86" Components of the Program 1. Extragalactic Distance Database" 2. Tip of the Red Giant Branch distances" 3. Luminosity-Linewidth (TF) distances" - HI profiles" - WISE photometry" " 4. Cosmicflows-2 distance compilation" 5. Modeling Absolute calibration Pop I: " HST Key Project Cepheid -
Arxiv:2006.03721V1 [Astro-Ph.CO] 5 Jun 2020 According to Tully Et Al
2018AstBu..73..124K Surveying the Local Supercluster Plane∗ O. G. Kashibadze∗∗,1 I. D. Karachentsev,1 and V. E. Karachentseva2 1Special Astrophysical Observatory, Russian Academy of Sciences, Nizhnii Arkhyz, 369167 Russia 2Main Astronomical Observatory, National Academy of Sciences of Ukraine, Kyiv, 03143 Ukraine We investigate the distribution and velocity field of galaxies situated in a band of 100 by 20 degrees centered on M 87 and oriented along the Local supercluster plane. Our sample amounts 2158 galaxies with radial velocities less than 2000 km s−1. Of them, 1119 galax- ies (52%) have distance and peculiar velocity estimates. About 3/4 of early-type galaxies are concentrated within the Virgo cluster core, most of the late-type galaxies in the band locate outside the virial radius. Distribution of gas-rich dwarfs with MHI > M∗ looks to be insensitive to the Virgo cluster presence. Among 50 galaxy groups in the equatorial su- percluster band 6 groups have peculiar velocities about 500{1000 km s−1 comparable with virial motions in rich clusters. The most cryptic case is a flock of nearly 30 galaxies around NGC 4278 (Coma I cloud), moving to us with the mean peculiar velocity of −840 km s−1. This cloud (or filament?) resides at a distance of 16.1 Mpc from us and approximately 5 Mpc away from the Virgo center. Galaxies around Virgo cluster exhibit Virgocentric infall with an amplitude of about 500 km s−1. Assuming the spherically symmetric radial infall, we estimate the radius of the zero-velocity surface to be R0 = (7:0 ± 0:3) Mpc that yields 14 the total mass of Virgo cluster to be (7:4 ± 0:9) × 10 M in tight agreement with its virial mass estimates. -
14. Absolute Revolution of the Superuniverses
Chapter 14 Absolute Revolution of the Superuniverses We are located near Uversa at the gravitational center of Orvonton. Uversa and the other six capitals of the superuniverses are located on the same orbital path about Paradise. There should be no relative velocity between these capitals, since they revolve as a single unit. There is a circular structure with a diameter of 18 million light-years in which galaxies have observed redshift velocities of zero. This circular structure passes directly over our location. Paradise is located at the center of this circular structure. There is a dramatic increase in the density of galaxies per unit of volume in this circular orbit. About 14 percent of all objects within 5-36 million light-years are found within the tight confines of this zero velocity orbit, almost half of which is directly visible. The density of galaxies in this orbital region is 289 times greater than it is in general. This is comparable to the difference between the mass densities of a gas and a solid. This positively identifies the existence of the central core of superuniverse space level as consisting of the galaxies in this zero velocity orbit. The angular velocity of the counterclockwise revolution of the superuniverses has been theoretically calculated at . The theoretical orbital velocity can be calculated from this using a distance of 9 Mly to Paradise. This theoretical orbital velocity in conjunction with the clockwise rotation of the first outer space level substantially explains the peculiar velocity of the Local Sheet, which has recently been found by astrophysicists. -
Arxiv:2108.10775V1 [Astro-Ph.HE] 24 Aug 2021
ICRC 2021 THE ASTROPARTICLE PHYSICS CONFERENCE ONLINE ICRC 2021Berlin | Germany THE ASTROPARTICLE PHYSICS CONFERENCE th Berlin37 International| Germany Cosmic Ray Conference 12–23 July 2021 Cosmographic model of the astroparticle skies Jonathan Biteau,0,∗ Sullivan Marafico,0 Younes Kerfis0 and Olivier Deligny0 0Université Paris-Saclay, CNRS/IN2P3, IJCLab, 91405 Orsay, France E-mail: [email protected] Modeling the extragalactic astroparticle skies involves reconstructing the 3D distribution of the most extreme sources in the Universe. Full-sky tomographic surveys at near-infrared wavelengths have already enabled the astroparticle community to bind the density of sources of astrophysical neutrinos and ultra-high cosmic rays (UHECRs), constrain the distribution of binary black-hole mergers and identify some of the components of the extragalactic gamma-ray background. This contribution summarizes the efforts of cleaning and complementing the catalogs developed by the gravitational-wave and near-infrared communities, in order to obtain a cosmographic view on stellar mass ("∗) and star formation rate (SFR). Unprecedented cosmography is offered by a sample of about 400,000 galaxies within 350 Mpc, with a 50-50 ratio of spectroscopic and photometric distances, "∗, SFR and corrections for incompleteness with increasing distance and decreasing Galactic latitude. The inferred 3D distribution of "∗ and SFR is consistent with Cosmic Flows. The "∗ and SFR densities converge towards values compatible with deep-field observations beyond 100 Mpc, suggesting a close-to-isotropic distribution of more distant sources. In addition to highlighting relevant applications for the four astroparticle communities, this contribution explores the distribution of 퐵-fields at Mpc scales deduced from the 3D distribution of matter, which is believed to be crucial in shaping the ultra-high-energy sky. -
Astronomy in Finland Are Summarized in Table 1
AASTRONOMYSTRONOMY ININ FFINLANDINLAND AN OVERVIEW IS GIVEN OF ASTRONOMICAL RESEARCH IN FINLAND. THERE ARE THREE INSTITUTES DEVOTED TO ASTRONOMY IN GENERAL, AT THE UNIVERSITIES OF HELSINKI, OULU, AND TURKU, AND A RADIO ASTRONOMY OBSERVATORY AT THE HELSINKI UNIVERSITY OF TECHNOLOGY. IN ADDITION, SOLAR SYSTEM RESEARCH WITH SPACE-BORNE INSTRUMENTATION IS BEING CARRIED OUT BY THE PHYSICS DEPARTMENTS AT THE UNIVERSITIES OF HELSINKI AND TURKU, AND BY THE FINNISH METEOROLOGICAL INSTITUTE. KALEVI MATTILA1, MERJA TORNIKOSKI2, ILKKA TUOMINEN3, ESKO VALTAOJA4 1OBSERVATORY, UNIVERSITY OF HELSINKI; 2METSÄHOVI RADIO OBSERVATORY; 3ASTRONOMY DIVISION, UNIVERSITY OF OULU; 4TUORLA OBSERVATORY INLAND HAS A LONG TRADITION Professor’s chair in 1828, and Argelander international enterprise in Astronomy so far, in Astronomy. The first was appointed its first holder. Based on the photographic “Carte du Ciel” survey, University in Finland, the accurate proper motions from his own started with the participation of Helsinki Academy of Turku (Åbo), was meridian circle observations in Turku and (Anders Donner) and seventeen other lead- founded in 1640, and earlier data from others, Argelander pub- ing observatories around the world. The FAstronomy was taught there from the very lished in 1837 a famous study on the deter- celestial mechanics tradition was continued F th beginning. The first Finnish scientist to rise mination of the Solar Apex. However, with- at the beginning of the 20 century by Karl to world fame was the astronomer and math- in the same year he moved to Bonn where he F. Sundman whose works on the three-body ematician Anders Lexell who was appoint- founded a new observatory and rose to problem brought him international fame. -
Mapping the Local Cosmic Web: Dark Matter Map Reveals Hidden Bridges Between Galaxies 25 May 2021
Mapping the local cosmic web: Dark matter map reveals hidden bridges between galaxies 25 May 2021 "Ironically, it's easier to study the distribution of dark matter much further away because it reflects the very distant past, which is much less complex," said Donghui Jeong, associate professor of astronomy and astrophysics at Penn State and a corresponding author of the study. "Over time, as the large-scale structure of the universe has grown, the complexity of the universe has increased, so it is inherently harder to make measurements about An international team of researchers has produced a dark matter locally." map of the dark matter within the local universe, using a model to infer its location due to its gravitational Previous attempts to map the cosmic web started influence on galaxies (black dots). These density with a model of the early universe and then maps--each a cross section in different simulated the evolution of the model over billions of dimensions--reproduce known, prominent features of the universe (red) and also reveal smaller filamentary years. However, this method is computationally features (yellow) that act as hidden bridges between intensive and so far has not been able to produce galaxies. The X denotes the Milky Way galaxy and results detailed enough to see the local universe. In arrows denote the motion of the local universe due to the new study, the researchers took a completely gravity. Credit: Hong et. al., Astrophysical Journal different approach, using machine learning to build a model that uses information about the distribution and motion of galaxies to predict the distribution of dark matter.