A Compact Endfire Radiation Antenna Based on Spoof Surface Plasmon Polaritons in Wide Bandwidth
Total Page:16
File Type:pdf, Size:1020Kb
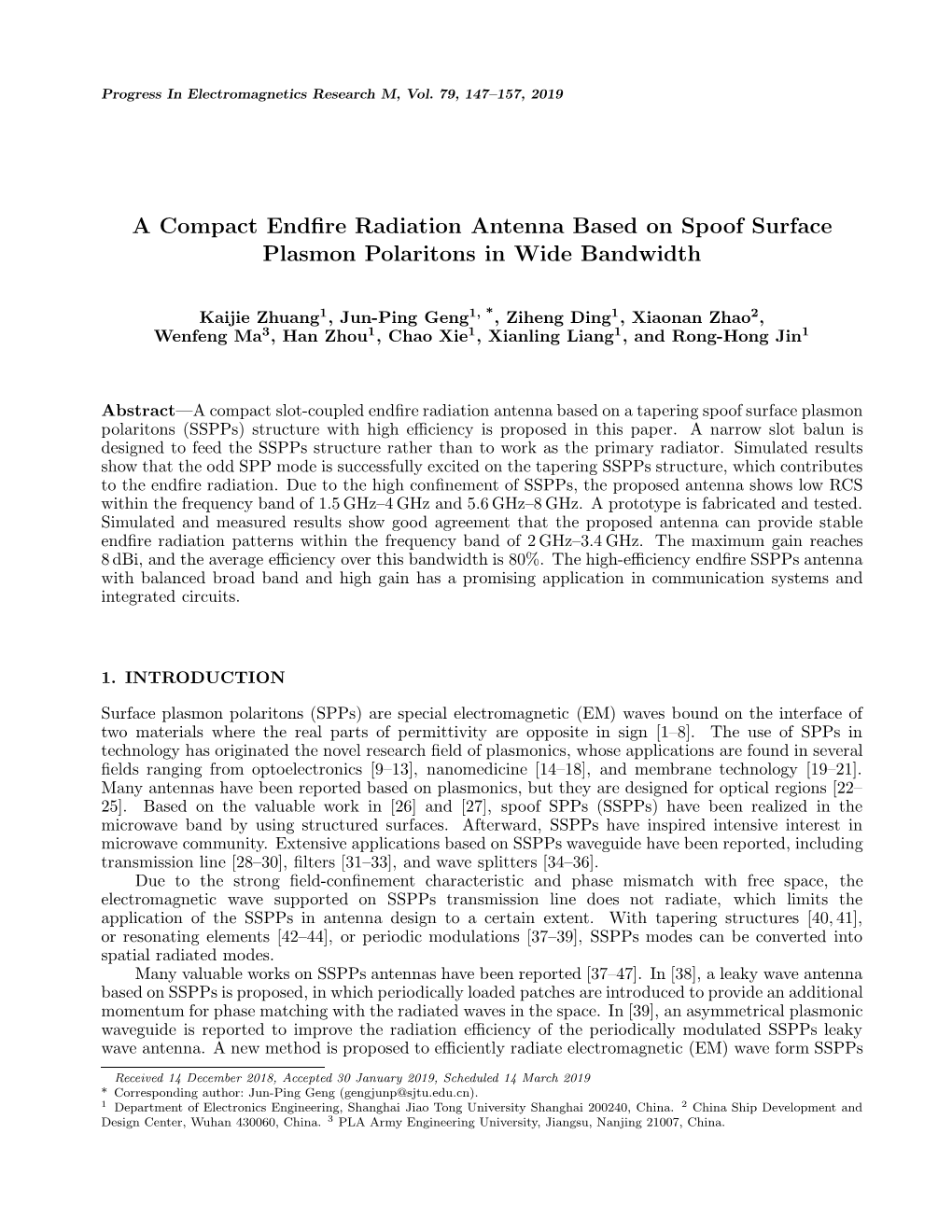
Load more
Recommended publications
-
A Spoof Surface Plasmon Polaritons (Sspps) Based Dual-Band-Rejection Filter with Wide Rejection Bandwidth
sensors Article A Spoof Surface Plasmon Polaritons (SSPPs) Based Dual-Band-Rejection Filter with Wide Rejection Bandwidth Ehsan Farokhipour 1 , Mohammad Mehrabi 1,† , Nader Komjani 1,* and Can Ding 2 1 Department of Electrical Engineering, Iran University of Science and Technology, Tehran 1684613114, Iran; [email protected] (E.F.); [email protected] (M.M.) 2 Global Big Data Technologies Centre, University of Technology Sydney, Sydney, NSW 2007, Australia; [email protected] * Correspondence: [email protected] † Current address: Division of Micro and Nanosystems, KTH Royal Institute of Technology, 11428 Stockholm, Sweden Received: 13 November 2020; Accepted: 16 December 2020; Published: 19 December 2020 Abstract: This paper presents a novel single-layer dual band-rejection-filter based on Spoof Surface Plasmon Polaritons (SSPPs). The filter consists of an SSPP-based transmission line, as well as six coupled circular ring resonators (CCRRs) etched among ground planes of the center corrugated strip. These resonators are excited by electric-field of the SSPP structure. The added ground on both sides of the strip yields tighter electromagnetic fields and improves the filter performance at lower frequencies. By removing flaring ground in comparison to prevalent SSPP-based constructions, the total size of the filter is significantly decreased, and mode conversion efficiency at the transition from co-planar waveguide (CPW) to the SSPP line is increased. The proposed filter possesses tunable rejection bandwidth, wide stop bands, and a variety of different parameters to adjust the forbidden bands and the filter’s cut-off frequency. To demonstrate the filter tunability, the effect of different elements like number (n), width (WR), radius (RR) of CCRRs, and their distance to the SSPP line (yR) are surveyed. -
Experimental Measurements of Fundamental and High-Order Spoof Surface Plasmon Polariton Modes on Ultrathin Metal Strips
Experimental measurements of fundamental and high-order spoof surface plasmon polariton modes on ultrathin metal strips Hong Xiang1, Qiang Zhang2, Jiwang Chai1, Fei Fei Qin2, Jun Jun Xiao2, and Dezhuan Han 1* 1 Department of Applied Physics, Chongqing University, Chongqing 400044, China 2 College of Electronic and Information Engineering, Shenzhen Graduate School, Harbin Institute of Technology, Shenzhen 518055, China *E-mail: [email protected] Abstract Propagation of spoof surface plasmon polaritons (spoof SPPs) on comb-shaped ultrathin metal strips made of aluminum foil and printed copper circuit are studied experimentally and numerically. With a near field scanning technique, electric field distributions on these metal strips are measured directly. The dispersion curves of spoof SPPs are thus obtained by means of Fourier transform of the field distributions in the real space for every frequency. Both fundamental and second order modes are investigated and the measured dispersions agree well with numerical ones calculated by the finite element method. Such direct measurements of the near field characteristics provide complete information of these spoof SPPs, enabling full exploitation of their properties associated with the field confinement in a subwavelength scale. Keywords: Perfect conductor; Spoof surface plasmon polariton; Near-field scanning Introduction Surface plasmon polariton (SPP) is a kind of surface wave propagating along the metal-dielectric interface with wavelengths smaller than that of the incident wave in free space [1,2], which is a consequence of coupling in between electromagnetic (EM) waves and collective oscillations of free electrons in metal. With the subwavelength nature, the EM field of SPP decays exponentially in the normal direction and exhibits strong confinement. -
Surface Electromagnetic Waves on Structured Perfectly Conducting Surfaces
UC Irvine UC Irvine Previously Published Works Title Surface electromagnetic waves on structured perfectly conducting surfaces Permalink https://escholarship.org/uc/item/75b3f673 Author Maradudin, AA Publication Date 2014 DOI 10.1016/B978-0-444-59526-3.00007-0 License https://creativecommons.org/licenses/by/4.0/ 4.0 Peer reviewed eScholarship.org Powered by the California Digital Library University of California CHAPTER Surface Electromagnetic Waves on Structured Perfectly Conducting Surfaces 7 Alexei A. Maradudin Research Professor, Physics and Astronomy School of Physical Sciences, University of California, Irvine, CA, USA A planar interface between a dielectric medium, e.g. vacuum, and a perfect conductor does not support a surface electromagnetic wave. This is easily seen. Let us consider a system consisting of vacuum in the region x3 > 0. We assume that a p-polarized electromagnetic wave of frequency ω is propagating in the x1 direction along the planar surface of a semi-infinite perfect conductor that occupies the region x3 < 0. The magnetic field in the vacuum region x3 > 0, H(x; t) = (0, H2(x1, x3|ω), 0) exp (−iωt), had only a single nonzero component. The amplitude H2(x1, x3|ω) satisfies the equation ∂2 ∂2 ω2 + + H (x , x |ω) = 0, (7.1) ∂ 2 ∂ 2 2 2 1 3 x1 x3 c together with the boundary condition ∂ H2(x1, x3|ω) = 0(7.2) ∂x 3 x3=0 on the surface of the perfect conductor. A solution of Eq. (7.1) that vanishes as x3 →∞is H2(x1, x3|ω) = A exp[ikx1 − β0(k)x3], (7.3) where 1 [k2 − (ω/c)2] 2 k2 >(ω/c)2, β0(k) = 1 (7.4) −i[(ω/c)2 − k2] 2 k2 <(ω/c)2. -
Spoof Surface Plasmon Polaritons Supported by Ultrathin Corrugated Metal Strip and Their Applications
Nanotechnol Rev 2015; 4(3): 239–258 Review Xi Gao and Tie Jun Cui* Spoof surface plasmon polaritons supported by ultrathin corrugated metal strip and their applications Abstract: In this review, we present a brief introduction Attributing to remarkable features and huge application on the spoof surface plasmons supported on corrugated potentials [3–10], SPPs have attracted extensive attentions metallic plates with nearly zero thickness. We mainly and have been intensively investigated. At optical frequen- focus on the propagation characteristics of spoof surface cies, metals behave like plasmas with negative permittiv- plasmon polaritons (SPPs), excitation of planar SPPs, and ity, which makes SPPs be highly confined to the interface several plasmonic devices including the bending wave- of metal and air (or metal and dielectric) and propagate guide, Y-shaped beam splitter, frequency splitter, and fil- along the surface. SPPs can overcome diffraction limit ter. These devices are designed and fabricated with either and realize miniaturized photonic components and inte- planar or conformal plasmonic metamaterials, which are grated circuits due to their highly localized feature, which validated by both full-wave simulations and experiments, makes it widely used in nano-photonics and optoelectron- showing high performance. We also demonstrate that an ics [4, 11–14]. However, as the frequency goes downward to ultrathin textured metallic disk can support multipolar microwave and terahertz regions, the natural SPPs do not spoof localized surface plasmons, either with straight or exist on smooth metal surfaces because of infinite dielec- curved grooves, from which the Fano resonances are also tric constant of metal [1]. Instead, Sommerfeld or Zenneck observed. -
Microwave Spoof Surface Plasmon Polariton-Based Sensor for Ultrasensitive Detection of Liquid Analyte Dielectric Constant
sensors Article Microwave Spoof Surface Plasmon Polariton-Based Sensor for Ultrasensitive Detection of Liquid Analyte Dielectric Constant Ivana Podunavac * , Vasa Radonic , Vesna Bengin and Nikolina Jankovic BioSense Institute, University of Novi Sad, Dr Zorana Djindjica 1, 21000 Novi Sad, Serbia; [email protected] (V.R.); [email protected] (V.B.); [email protected] (N.J.) * Correspondence: [email protected] Abstract: In this paper, a microwave microfluidic sensor based on spoof surface plasmon polaritons (SSPPs) was proposed for ultrasensitive detection of dielectric constant. A novel unit cell for the SSPP structure is proposed and its behaviour and sensing potential analysed in detail. Based on the proposed cell, the SSPP microwave structure with a microfluidic reservoir is designed as a multilayer configuration to serve as a sensing platform for liquid analytes. The sensor is realized using a combination of rapid, cost-effective technologies of xurography, laser micromachining, and cold lamination bonding, and its potential is validated in the experiments with edible oil samples. The results demonstrate high sensitivity (850 MHz/epsilon unit) and excellent linearity (R2 = 0.9802) of the sensor, which, together with its low-cost and simple fabrication, make the proposed sensor an excellent candidate for the detection of small changes in the dielectric constant of edible oils and other liquid analytes. Keywords: microwave sensor; spoof surface plasmon polariton (SSPP); permittivity sensing; edi- Citation: Podunavac, I.; Radonic, V.; ble oils Bengin, V.; Jankovic, N. Microwave Spoof Surface Plasmon Polariton-Based Sensor for Ultrasensitive Detection of Liquid 1. Introduction Analyte Dielectric Constant. Sensors Due to their possibility for real-time, non-contact and non-invasive measurements, 2021, 21, 5477. -
Tunable Reflectionless Absorption of Electromagnetic Waves in a Plasma- Metamaterial Composite Structure
Plasma Sources Science and Technology ACCEPTED MANUSCRIPT Tunable reflectionless absorption of electromagnetic waves in a plasma- metamaterial composite structure To cite this article before publication: Nolan Uchizono et al 2020 Plasma Sources Sci. Technol. in press https://doi.org/10.1088/1361- 6595/aba489 Manuscript version: Accepted Manuscript Accepted Manuscript is “the version of the article accepted for publication including all changes made as a result of the peer review process, and which may also include the addition to the article by IOP Publishing of a header, an article ID, a cover sheet and/or an ‘Accepted Manuscript’ watermark, but excluding any other editing, typesetting or other changes made by IOP Publishing and/or its licensors” This Accepted Manuscript is © 2020 IOP Publishing Ltd. During the embargo period (the 12 month period from the publication of the Version of Record of this article), the Accepted Manuscript is fully protected by copyright and cannot be reused or reposted elsewhere. As the Version of Record of this article is going to be / has been published on a subscription basis, this Accepted Manuscript is available for reuse under a CC BY-NC-ND 3.0 licence after the 12 month embargo period. After the embargo period, everyone is permitted to use copy and redistribute this article for non-commercial purposes only, provided that they adhere to all the terms of the licence https://creativecommons.org/licences/by-nc-nd/3.0 Although reasonable endeavours have been taken to obtain all necessary permissions from third parties to include their copyrighted content within this article, their full citation and copyright line may not be present in this Accepted Manuscript version. -
Recent Advancements in Surface Plasmon Polaritons- Plasmonics in Subwavelength Structures at Microwave and Terahertz Regime
Accepted Manuscript Recent advancements in surface plasmon polaritons- plasmonics in subwavelength structures at microwave and terahertz regime Rana Sadaf Anwar, HuanSheng Ning, LingFeng Mao PII: S2352-8648(17)30199-2 DOI: 10.1016/j.dcan.2017.08.004 Reference: DCAN 98 To appear in: Digital Communications and Networks Received Date: 20 June 2017 Revised Date: 2352-8648 2352-8648 Accepted Date: 9 August 2017 Please cite this article as: R.S. Anwar, H. Ning, L. Mao, Recent advancements in surface plasmon polaritons- plasmonics in subwavelength structures at microwave and terahertz regime, Digital Communications and Networks (2017), doi: 10.1016/j.dcan.2017.08.004. This is a PDF file of an unedited manuscript that has been accepted for publication. As a service to our customers we are providing this early version of the manuscript. The manuscript will undergo copyediting, typesetting, and review of the resulting proof before it is published in its final form. Please note that during the production process errors may be discovered which could affect the content, and all legal disclaimers that apply to the journal pertain. Recent advancements in Surface Plasmon Polaritons- ACCEPTED MANUSCRIPT Plasmonics in Subwavelength Structures at Microwave and Terahertz regime Rana Sadaf Anwar*, HuanSheng Ning, LingFeng Mao School of computer and communication Engineering, University of Science and Technology, Beijing, China, 100083 Abstract A review of recent investigational studies has been presented in this paper of exciting the surface plasmon polaritons (SPPs) in microwave (MW) and terahertz (THz) regime by using subwavelength corrugated patterns on conducting or metal surfaces. This article also outlines the significance of SPPs microstrip (MS) structures at microwave and terahertz frequencies, compared to the conventional MS transmission lines (TL) to tackle the key challenges of high gain, broader bandwidth, compactness, TL losses and signal integrity in high-end electronics devices. -
Integrated Spoof Plasmonic Circuits ⇑ Jingjing Zhang, Hao-Chi Zhang, Xin-Xin Gao, Le-Peng Zhang, Ling-Yun Niu, Pei-Hang He, Tie-Jun Cui
Science Bulletin 64 (2019) 843–855 Contents lists available at ScienceDirect Science Bulletin journal homepage: www.elsevier.com/locate/scib Review Integrated spoof plasmonic circuits ⇑ Jingjing Zhang, Hao-Chi Zhang, Xin-Xin Gao, Le-Peng Zhang, Ling-Yun Niu, Pei-Hang He, Tie-Jun Cui State Key Laboratory of Millimeter Waves, Southeast University, Nanjing 210096, China article info abstract Article history: Using a metamaterial consisting of metals with subwavelength surface patterning, one can mimic surface Received 30 November 2018 plasmon polaritons (SPPs) and achieve surface waves with subwavelength confinement at microwave Received in revised form 21 January 2019 and terahertz frequencies, thus bringing most of the advantages associated with the optical SPPs to lower Accepted 23 January 2019 frequencies. Due to the properties of strong field confinement and high local field intensity, spoof SPPs Available online 2 February 2019 have demonstrated the improved performance for data transmission and device miniaturization in an intensively integrated environment. The distinctive abilities, such as suppression of transmission loss Keywords: and bending loss, and increase of signal integrity, make spoof SPPs a promising candidate for future gen- Spoof surface plasmons eration of electronic circuits and electromagnetic systems. This article reviews the progress in spoof SPPs Metamaterial Electromagnetic system with a special focus on their applications in circuits from transmission lines to passive and active devices Integrated circuits in microwave and terahertz regimes. The integration of versatile spoof SPP devices on a single platform, which is compatible with established electronic circuits, is also discussed. Ó 2019 Science China Press. Published by Elsevier B.V. and Science China Press. -
Scattering of Spoof Surface Plasmon Polaritons in Defect-Rich Thz Waveguides Received: 14 January 2019 Andreas K
www.nature.com/scientificreports OPEN Scattering of spoof surface plasmon polaritons in defect-rich THz waveguides Received: 14 January 2019 Andreas K. Klein1, Alastair Basden2, Jonathan Hammler1, Luke Tyas2, Michael Cooke1, Accepted: 24 March 2019 Claudio Balocco1, Dagou Zeze1, John M. Girkin 2 & Andrew Gallant1 Published: xx xx xxxx We report on the frst observation of ‘Spoof’ Surface Plasmon Polariton (SPP) scattering from surface defects on metal-coated 3D printed, corrugated THz waveguiding surfaces. Surface defects, a result of the printing process, are shown to assist the direct coupling of the incident free-space radiation into a spoof SPP wave; removing the need to bridge the photon momentum gap using knife-edge or prism coupling. The free space characteristics, propagation losses and confnement of the spoof SPPs to the surface are measured, and the results are compared to fnite-diference time domain simulations. Angular resolved THz spectroscopy measurements reveal the scattering patterns from surfaces and are compared with Mie theory, taking into account the shortened wavelength of the photons in their bound SPP state compared to their free space wavelength. These results confrm yet another similarity between the properties of THz spoof SPPs and their natural, non-spoof, counterparts at optical and infrared frequencies which also, unexpectedly, adds functionality to the structures. Te feld of plasmonics ofers the possibility for sub-wavelength manipulation of electromagnetic radiation which can lead to more compact and efcient optical devices or optical circuits1–3. Te concept of spoof-SPPs, where a surface structured on a sub-wavelength scale supports modes with cut-of frequencies determined by the geome- try, allows the mimicking of SPPs and extended plasmonics to virtually all frequencies4,5. -
Spoof Surface Plasmon Based Planar Thz Sensor System Using Dumbbell Shaped Unit Cell
2018 International Symposium on Antennas and Propagation (ISAP 2018) [WeE2-5] October 23~26, 2018 / Paradise Hotel Busan, Busan, Korea Spoof Surface Plasmon based Planar THz Sensor System using Dumbbell Shaped Unit Cell M Jaleel Akhtar, Nilesh K Tiwari, Surya P Singh Department of Electrical Engineering, Indian Institute of Technology Kanpur, India Abstract - A spoof surface plasmon polariton (SSPP) optimized in the microwave frequency region for obtaining transmission line based THz sensor using a perforated the desired characteristics. dumbbell shaped unit cell is presented. The proposed SSPP structure possesses better confinement ability as compared to In this paper, the dumbbell shaped unit cell geometry is the conventional microstrip line in the THz frequency regime proposed to design the microstrip fed SSPP structure with which actually helps to realize the THz sensor with improved the underlying ground in the microwave frequency band. The sensitivity. The designed SSPPs based structure is having under proposed dumbbell shaped SSPP structure with under layer layer ground in order to obtain better conversion between ground is proven to be quite compact possessing improved conventional microstrip to SSPP structure due to efficient impedance matching between them in the microwave and THz confinement ability as compared to that of the conventional frequency band. To design the THz dielectric sensor using the microstrip lines. This property of proposed SSPP structure is proposed highly confined SSPP transmission an appropriate exploited here to design the THz/ microwave sensor with capacitive slot on the dumbbell cells is created. Design and improved sensitivity by creating the capacitive slot on the optimization of the proposed SSPP based resonant structure is dumbbell unit cell. -
Spoof Surface Plasmon Waveguide Forces
Spoof surface plasmon waveguide forces The Harvard community has made this article openly available. Please share how this access benefits you. Your story matters Citation Woolf, David, Mikhail A. Kats, and Federico Capasso. 2014. “Spoof Surface Plasmon Waveguide Forces.” Optics Letters 39 (3): 517. https://doi.org/10.1364/ol.39.000517. Citable link http://nrs.harvard.edu/urn-3:HUL.InstRepos:41371345 Terms of Use This article was downloaded from Harvard University’s DASH repository, WARNING: This file should NOT have been available for downloading from Harvard University’s DASH repository. February 1, 2014 / Vol. 39, No. 3 / OPTICS LETTERS 517 Spoof surface plasmon waveguide forces David Woolf, Mikhail A. Kats, and Federico Capasso* School of Engineering and Applied Sciences, Harvard University, Cambridge, Massachussettes 02138, USA *Corresponding author: [email protected] Received October 29, 2013; accepted November 16, 2013; posted December 13, 2013 (Doc. ID 200260); published January 23, 2014 Spoof surface plasmons (SP) are SP-like waves that propagate along metal surfaces with deeply sub-wavelength corrugations and whose dispersive properties are determined primarily by the corrugation dimensions. Two parallel corrugated surfaces separated by a sub-wavelength dielectric gap create a “spoof” analog of the plasmonic metal–insulator–metal waveguides, dubbed a “spoof-insulator-spoof” (SIS) waveguide. Here we study the optical forces generated by the propagating “bonding” and “anti-bonding” waveguide modes of the SIS geometry and the role that surface structuring plays in determining the modal properties. By changing the dimensions of the grooves, strong attractive and repulsive optical forces between the surfaces can be generated at nearly any frequency. -
Amplifying Evanescent Waves by Dispersion- Induced Plasmons: Defying the Materials Limitation of Superlens
Amplifying Evanescent Waves by Dispersion- induced Plasmons: Defying the Materials Limitation of Superlens Tie-Jun Huang, Li-Zheng Yin, Jin Zhao, Chao-Hai Du, Pu-Kun Liu* State Key Laboratory of Advanced Optical Communication Systems and Networks, Department of Electronics, Peking University, Beijing, 100871, China *Correspondence to [email protected] Breaking the diffraction limit is always an appealing topic due to the urge for a better imaging resolution in almost all areas. As an effective solution, the superlens based on the plasmonic effect can resonantly amplify evanescent waves, and achieve subwavelength resolution. However, the natural plasmonic materials, within their limited choices, usually have inherit high losses and are only available from the infrared to visible wavelengths. In this work, we theoretically and experimentally demonstrate that the arbitrary materials, even air, can be used to enhance evanescent waves and build low loss superlens with at the desired frequency. The operating mechanisms reside in the dispersion-induced effective plasmons in a bounded waveguide structure. Based on this, we construct the hyperbolic metamaterials and experimentally verified its validity in the microwave range by the directional propagation and imaging with a resolution of 0.087λ. We also demonstrate that the imaging potential can be extended to terahertz and infrared bands. The proposed method not only break the conventional barriers of plasmon- based lenses, but also bring possibilities in applications based on the enhancing evanescent waves from microwave to infrard wavelengths, such as ultrasensitive optics, spontaneous emission, light beam steering. 1 Introduction Imaging with the unlimited resolution has been an intriguing dream of scientists for a few centuries.