Single Molecule Dynamics of Dishevelled at the Plasma Membrane and Wnt Pathway Activation
Total Page:16
File Type:pdf, Size:1020Kb
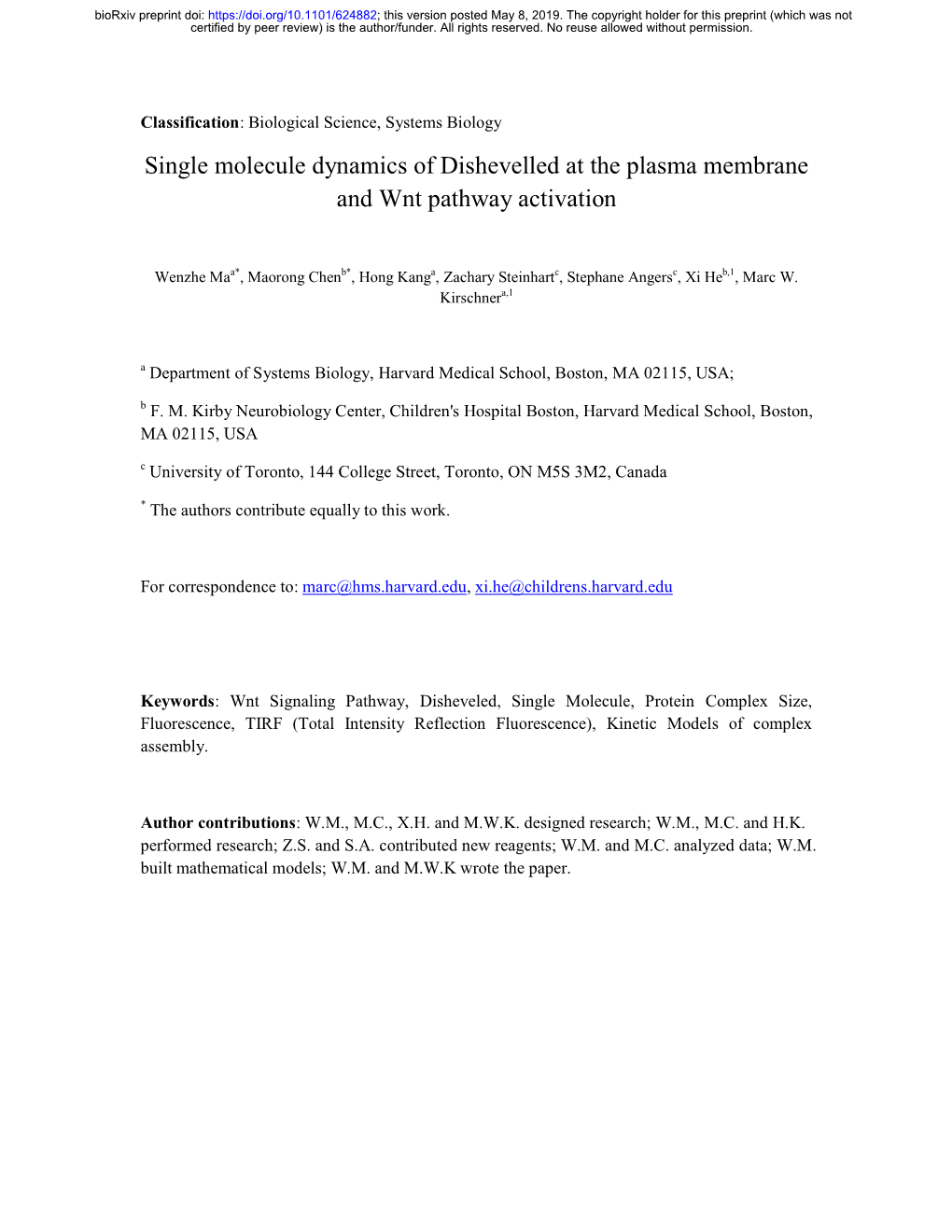
Load more
Recommended publications
-
THE GENOMIC STRUCTURE of the ZEBRAFISH Wnt8b GE NE
THE GENOMIC STRUCTURE OF THE ZEBRAFISH wnt8b GENE by Yvonne Marie Beckham Department of Zoology Submitted in partial fulfilment of the requirements for the degree of Master of Science Faculty of Graduate Studies The University of Western Ontario London, Ontario December 1997 O Yvonne Marie Beckham, 1997 National Library Bibliothèque nationale du Canada Acquisitions and Acquisitions et Bibliographie Services seMces bibliographiques 395 Weüingtaci Street 395. Ne Wellington OttawaON K1AON4 OttawaON K1AW canada canada The author has granted a non- L'auteur a accordé une licence non exclusive licence allowing the exclusive permettant à la National Lhrary of Canada to Bibliothèque nationale du Canada de reproduce, loan, distribute or sell reproduire, prêter, districbuer ou copies of this thesis in microform, vendre des copies de cette thèse sous paper or electronic formats. la forme de microfiche/nlm, de reproduction sur papier ou sur fonnat électronique. The author retains ownership of the L'auteur conserve la propriété du copyright in this thesis. Neither the droit d'auteur qui protège cette thèse. thesis nor substantial extracts fiom it Ni la thèse ni des extraits substantiels may be printed or otherwise de celle-ci ne doivent être imprimés reproduced without the author's ou autrement reproduits sans son permission. autorisation. ABSTRACT Screening of a zebrafish genomic library to identify the prornoter elements of the zebrafish ivnr8b gene resulted in the isolation of two clones. p8b-3H and p8b-7A. Southem blot analysis demonstrated that clone p8b- 3H contained the 3' portion of the cDNA. p8b-7A showed only weak hybridization to the wnr8b cDNA used to initially isolate this clone. -
Regulation of Dishevelled DEP Domain Swapping by Conserved Phosphorylation Sites
Regulation of Dishevelled DEP domain swapping by conserved phosphorylation sites Gonzalo J. Beitiaa,1, Trevor J. Rutherforda,1, Stefan M. V. Freunda, Hugh R. Pelhama, Mariann Bienza, and Melissa V. Gammonsa,2 aMedical Research Council Laboratory of Molecular Biology, Cambridge Biomedical Campus, Cambridge, CB2 0QH, United Kingdom Edited by Roeland Nusse, Stanford University School of Medicine, Stanford, CA, and approved May 13, 2021 (received for review February 20, 2021) Wnt signals bind to Frizzled receptors to trigger canonical and with these effectors even if present at a low cellular concentra- noncanonical signaling responses that control cell fates during tion (1, 3, 12). animal development and tissue homeostasis. All Wnt signals are The DEP domain is a small globular domain composed of three relayed by the hub protein Dishevelled. During canonical (β-catenin– α-helices and a flexible hinge loop between the first (H1) and sec- dependent) signaling, Dishevelled assembles signalosomes via dy- ond helix (H2), which, in the monomeric configuration, folds back namic head-to-tail polymerization of its Dishevelled and Axin (DIX) on itself to form a prominent “DEP finger” that is responsible domain, which are cross-linked by its Dishevelled, Egl-10, and Pleck- for binding to Frizzled (Fig. 1A) (13). DEP dimerization involves strin (DEP) domain through a conformational switch from monomer a highly unusual mechanism called “domain swapping” (14). During to domain-swapped dimer. The domain-swapped conformation of this process, H1 of one DEP monomer is exchanged with H1 from a DEP masks the site through which Dishevelled binds to Frizzled, im- reciprocal one through outward motions of the hinge loops, replacing plying that DEP domain swapping results in the detachment of Dish- intra- with intermolecular contacts. -
Systems Analysis Implicates WAVE2&Nbsp
JACC: BASIC TO TRANSLATIONAL SCIENCE VOL.5,NO.4,2020 ª 2020 THE AUTHORS. PUBLISHED BY ELSEVIER ON BEHALF OF THE AMERICAN COLLEGE OF CARDIOLOGY FOUNDATION. THIS IS AN OPEN ACCESS ARTICLE UNDER THE CC BY-NC-ND LICENSE (http://creativecommons.org/licenses/by-nc-nd/4.0/). PRECLINICAL RESEARCH Systems Analysis Implicates WAVE2 Complex in the Pathogenesis of Developmental Left-Sided Obstructive Heart Defects a b b b Jonathan J. Edwards, MD, Andrew D. Rouillard, PHD, Nicolas F. Fernandez, PHD, Zichen Wang, PHD, b c d d Alexander Lachmann, PHD, Sunita S. Shankaran, PHD, Brent W. Bisgrove, PHD, Bradley Demarest, MS, e f g h Nahid Turan, PHD, Deepak Srivastava, MD, Daniel Bernstein, MD, John Deanfield, MD, h i j k Alessandro Giardini, MD, PHD, George Porter, MD, PHD, Richard Kim, MD, Amy E. Roberts, MD, k l m m,n Jane W. Newburger, MD, MPH, Elizabeth Goldmuntz, MD, Martina Brueckner, MD, Richard P. Lifton, MD, PHD, o,p,q r,s t d Christine E. Seidman, MD, Wendy K. Chung, MD, PHD, Martin Tristani-Firouzi, MD, H. Joseph Yost, PHD, b u,v Avi Ma’ayan, PHD, Bruce D. Gelb, MD VISUAL ABSTRACT Edwards, J.J. et al. J Am Coll Cardiol Basic Trans Science. 2020;5(4):376–86. ISSN 2452-302X https://doi.org/10.1016/j.jacbts.2020.01.012 JACC: BASIC TO TRANSLATIONALSCIENCEVOL.5,NO.4,2020 Edwards et al. 377 APRIL 2020:376– 86 WAVE2 Complex in LVOTO HIGHLIGHTS ABBREVIATIONS AND ACRONYMS Combining CHD phenotype–driven gene set enrichment and CRISPR knockdown screening in zebrafish is an effective approach to identifying novel CHD genes. -
EGFR Confers Exquisite Specificity of Wnt9a-Fzd9b Signaling in Hematopoietic Stem Cell Development
bioRxiv preprint doi: https://doi.org/10.1101/387043; this version posted August 7, 2018. The copyright holder for this preprint (which was not certified by peer review) is the author/funder. All rights reserved. No reuse allowed without permission. Grainger, et al, 2018 EGFR confers exquisite specificity of Wnt9a-Fzd9b signaling in hematopoietic stem cell development Stephanie Grainger1, Nicole Nguyen1, Jenna Richter1,2, Jordan Setayesh1, Brianna Lonquich1, Chet Huan Oon1, Jacob M. Wozniak2,3,4, Rocio Barahona1, Caramai N. Kamei5, Jack Houston1,2, Marvic Carrillo-Terrazas3,4, Iain A. Drummond5,6, David Gonzalez3.4, Karl Willert#,¥,1, and David Traver¥,1,7. ¥co-corresponding authors: [email protected]; [email protected] #Lead contact 1Department of Cellular and Molecular Medicine, University of California, San Diego, La Jolla, California, 92037, USA. 2Biomedical Sciences Graduate Program, University of California, San Diego, La Jolla, California, 92037, USA. 3Skaggs School of Pharmacy and Pharmaceutical Science, University of California, San Diego, La Jolla, California, 92093, USA. 4Department of Pharmacology, University of California, San Diego, La Jolla, California, 92092 5Massachusetts General Hospital Nephrology Division, Charlestown, Massachusetts, 02129, USA. 6Harvard Medical School, Department of Genetics, Boston MA 02115 7Section of Cell and Developmental Biology, University of California, San Diego, La Jolla, California, 92037, USA. Running title: A mechanism for Wnt-Fzd specificity in hematopoietic stem cells Keywords: hematopoietic stem cell (HSC), Wnt, Wnt9a, human, zebrafish, Fzd, Fzd9b, FZD9, EGFR, APEX2 1 bioRxiv preprint doi: https://doi.org/10.1101/387043; this version posted August 7, 2018. The copyright holder for this preprint (which was not certified by peer review) is the author/funder. -
Identification and Characterization of the Rat DVL2 Gene Using
TurkJBiol 31(2007)81-86 ©TÜB‹TAK IdentificationandCharacterizationoftheRatDVL2GeneUsing BioinformaticTools LokmanVARIfiLI,OsmanÇEN DepartmentofBiology,FacultyofArtsandScience,HarranUniversity,fianl›urfa-TURKEY Received:02.10.2006 Abstract: WeidentifiedandcharacterizedtheratDVL2geneusingbioinformatics.Inadditiontothestructureandchromosomal localizationoftheratDVL2gene,thetranscribedandtranslatedproteinproductofthegenewasanalyzedinsilico.Resultss howed thattheratDVL2geneconsistsof15exonsandislocatedontheratgenomiccontigWGA1854.3onchromosome10.Database searchesusingtheratDVL2aminoacidsequenceasaqueryshowedanumberofhomologousproteinsequencesindifferentspecies, includingM.musculus,P.troglodytes,C.familiaris,H.sapiens,B.taurus,D.rerio,X.laevis,and T.nigroviridis.DAX,PDZsignaling, andDEP-conserveddomainstructureswereidentifiedwithintheratDVL2protein. KeyWords: Bioinformatics,DVL2,ratgenome,Wntsignaling BiyoinformatikMetodlarKullanarakS›çanDVL2GenininTan›mlanmas›veKarakterizasyonu Özet: Buçal›flmadabiyoinformatikyaklafl›mlarkullanaraks›çanDVL2geninitan›mlad›kvekarakterizeettik.S›çanDVL2genininyap› vekromozomallokalizasyonunaekolarakgeninkodlad›¤›proteindeinsilicoolarakanalizedildi.Sonuçlar›m›zagöres›çanDVL2geni 15eksondanoluflmuflve10.kromozomdakigenomikkontigWGA1854.3üzerindebulunmaktad›r.S›çanDVL2proteininin M. musculus,P.troglodytes,C.familiaris,H.sapiens,B.taurus,D.rerio,X.laevis ve T.nigroviridis türlerindekihomologlar›vebunlar aras›ndakihomolojioranlar›aminoasitduzeyindebelirlendi.RatDVL2proteinindeDAX,PDZ-SinyalizasyonveDEPkorunmufl domeynyap›lar›oldu¤ubelirlendi. -
Dishevelled Genes Mediate a Conserved Mammalian PCP
RESEARCH ARTICLE 1767 Development 133, 1767-1778 (2006) doi:10.1242/dev.02347 Dishevelled genes mediate a conserved mammalian PCP pathway to regulate convergent extension during neurulation Jianbo Wang1, Natasha S. Hamblet1,*, Sharayne Mark2, Mary E. Dickinson3,†, Brendan C. Brinkman4, Neil Segil5, Scott E. Fraser3, Ping Chen2, John B. Wallingford6 and Anthony Wynshaw-Boris1,§ The planar cell polarity (PCP) pathway is conserved throughout evolution, but it mediates distinct developmental processes. In Drosophila, members of the PCP pathway localize in a polarized fashion to specify the cellular polarity within the plane of the epithelium, perpendicular to the apicobasal axis of the cell. In Xenopus and zebrafish, several homologs of the components of the fly PCP pathway control convergent extension. We have shown previously that mammalian PCP homologs regulate both cell polarity and polarized extension in the cochlea in the mouse. Here we show, using mice with null mutations in two mammalian Dishevelled homologs, Dvl1 and Dvl2, that during neurulation a homologous mammalian PCP pathway regulates concomitant lengthening and narrowing of the neural plate, a morphogenetic process defined as convergent extension. Dvl2 genetically interacts with Loop-tail, a point mutation in the mammalian PCP gene Vangl2, during neurulation. By generating Dvl2 BAC (bacterial artificial chromosome) transgenes and introducing different domain deletions and a point mutation identical to the dsh1 allele in fly, we further demonstrated a high degree of conservation between Dvl function in mammalian convergent extension and the PCP pathway in fly. In the neuroepithelium of neurulating embryos, Dvl2 shows DEP domain-dependent membrane localization, a pre-requisite for its involvement in convergent extension. -
Recruitment of Adenomatous Polyposis Coli and B-Catenin to Axin-Puncta
Oncogene (2008) 27, 5808–5820 & 2008 Macmillan Publishers Limited All rights reserved 0950-9232/08 $32.00 www.nature.com/onc ORIGINAL ARTICLE Recruitment of adenomatous polyposis coli and b-catenin to axin-puncta MC Faux, JL Coates, B Catimel, S Cody, AHA Clayton, MJ Layton and AW Burgess Ludwig Institute for Cancer Research, Melbourne Tumour Biology Branch, Parkville, Victoria, Australia The adenomatous polyposis coli (APC)tumour suppressor coli (APC) form a complex that promotes the phos- is a multifunctional protein involved in the regulation of phorylation of b-catenin by glycogen synthase kinase-3-b Wnt signalling and cytoskeletal dynamics. Little is known (GSK3-b), which consequently targets b-catenin for about how APC controls these disparate functions. In this ubiquitination by bTrCP and destruction by the study, we have used APC- and axin-fluorescent fusion proteasome (Aberle et al., 1997; Orford et al., 1997; proteins to examine the interactions between these Kitagawa et al., 1999). Wnt stimulation and APC proteins and show that the functionally distinct popula- mutations disrupt the APC/axin complex and result in tions of APC are also spatially separate. Axin-RFP forms increased levels of b-catenin in the nucleus and cytoplasmic punctate structures, similar to endogenous cytoplasm (Munemitsu et al., 1995). b-Catenin is known axin puncta. Axin-RFP recruits b-catenin destruction to interact with members of the Tcf family of transcrip- complex proteins, including APC, b-catenin, glycogen tion factors to activate transcription of genes involved in synthase kinase-3-b (GSK3-b)and casein kinase-1- a proliferation and cellular transformation (Korinek (CK1-a). -
Palmitic Acid Effects on Hypothalamic Neurons
bioRxiv preprint doi: https://doi.org/10.1101/2021.08.03.454666; this version posted August 4, 2021. The copyright holder for this preprint (which was not certified by peer review) is the author/funder, who has granted bioRxiv a license to display the preprint in perpetuity. It is made available under aCC-BY-NC-ND 4.0 International license. Running title: Oleic and palmitic acid effects on hypothalamic neurons Concentration-dependent change in hypothalamic neuronal transcriptome by the dietary fatty acids: oleic and palmitic acids Fabiola Pacheco Valencia1^, Amanda F. Marino1^, Christos Noutsos1, Kinning Poon1* 1Department of Biological Sciences, SUNY Old Westbury, Old Westbury NY, United States ^Authors contributed equally to this work *Corresponding Author: Kinning Poon 223 Store Hill Rd Old Westbury, NY 11568, USA 1-516-876-2735 [email protected] bioRxiv preprint doi: https://doi.org/10.1101/2021.08.03.454666; this version posted August 4, 2021. The copyright holder for this preprint (which was not certified by peer review) is the author/funder, who has granted bioRxiv a license to display the preprint in perpetuity. It is made available under aCC-BY-NC-ND 4.0 International license. Abstract Prenatal high-fat diet exposure increases hypothalamic neurogenesis events in embryos and programs offspring to be obesity-prone. The molecular mechanism involved in these dietary effects of neurogenesis are unknown. This study investigated the effects of oleic and palmitic acids, which are abundant in a high-fat diet, on the hypothalamic neuronal transcriptome and how these changes impact neurogenesis events. The results show differential effects of low and high concentrations of oleic or palmitic acid treatment on differential gene transcription. -
PDZ Domain from Dishevelled — a Specificity Study Katarzyna Śmietana, Agnieszka Mateja, Artur Krężel and Jacek Otlewski*
Vol. 58, No. 2/2011 243–249 on-line at: www.actabp.pl Regular paper PDZ domain from Dishevelled — a specificity study Katarzyna Śmietana, Agnieszka Mateja, Artur Krężel and Jacek Otlewski* Faculty of Biotechnology, Department of Protein Engineering, University of Wrocław, Wrocław, Poland Intracellular signaling cascades induced by Wnt proteins play a key role in developmental processes and are im- It is still unclear what mechanism governs the activa- plicated in cancerogenesis. It is still unclear how the cell tion of the correct Wnt signaling branch. Dishevelled determines which of the three possible Wnt response (Dvl) proteins, the last shared link of the canonical and mechanisms should be activated, but the decision proc- non-canonical pathways, most likely play a key regulatory ess is most likely dependent on Dishevelled proteins. role in the signal distribution process (Habas & Dawid, Dishevelled family members interact with many diverse 2005; Itoh et al., 2005; Leonard & Ettensohn, 2007). This targets, however, molecular mechanisms underlying notion is further supported by the fact that the multi- these binding events have not been comprehensively tude of Dvl binding partners includes proteins that re- described so far. Here, we investigated the specificity of lay signaling to β-catenin pathways (axin (Li et al., 1999; the PDZ domain from human Dishevelled-2 using C-ter- Wharton, 2003), GBP/Frat (Li et al., 1999)), PCP (Rac1 minal phage display, which led us to identification of a (Fanto et al., 2000), Daam1 (Habas et al., 2001), strabis- 2+ leucine-rich binding motif strongly resembling the con- mus (Bastock et al., 2003)) and Ca (Gαo/Gαt (Liu et al., sensus sequence of a nuclear export signal. -
Roco Proteins and the Parkinson's Disease-Associated LRRK2
International Journal of Molecular Sciences Review Roco Proteins and the Parkinson’s Disease-Associated LRRK2 Jingling Liao 1,2,* and Quyen Q. Hoang 2,3,4,* 1 Department of Public Health, Wuhan University of Science and Technology School of Medicine, Wuhan 430081, China 2 Department of Biochemistry and Molecular Biology, Indiana University School of Medicine, Indianapolis, IN 46202, USA 3 Department of Neurology, Indiana University School of Medicine, Indianapolis, IN 46202, USA 4 Stark Neurosciences Research Institute, Indiana University School of Medicine, Indianapolis, IN 46202, USA * Correspondence: [email protected] (J.L.); [email protected] (Q.Q.H.); Tel.: +86-177-8646-6520 (J.L.); +1-317-274-4371 (Q.Q.H.) Received: 15 November 2018; Accepted: 14 December 2018; Published: 17 December 2018 Abstract: Small G-proteins are structurally-conserved modules that function as molecular on-off switches. They function in many different cellular processes with differential specificity determined by the unique effector-binding surfaces, which undergo conformational changes during the switching action. These switches are typically standalone monomeric modules that form transient heterodimers with specific effector proteins in the ‘on’ state, and cycle to back to the monomeric conformation in the ‘off’ state. A new class of small G-proteins called “Roco” was discovered about a decade ago; this class is distinct from the typical G-proteins in several intriguing ways. Their switch module resides within a polypeptide chain of a large multi-domain protein, always adjacent to a unique domain called COR, and its effector kinase often resides within the same polypeptide. As such, the mechanisms of action of the Roco G-proteins are likely to differ from those of the typical G-proteins. -
And Right-Sided Colon Cancer
Author Manuscript Published OnlineFirst on November 29, 2017; DOI: 10.1158/1541-7786.MCR-17-0483 Author manuscripts have been peer reviewed and accepted for publication but have not yet been edited. Multi-omics Approach Reveals Distinct Differences in Left- and Right-sided Colon Cancer Running title: Left- and Right-sided Colon Cancer Comparison Wangxiong Hu1*†, Yanmei Yang2*, Xiaofen Li1,3, Minran Huang1, Fei Xu1, Weiting Ge1†, Suzhan Zhang1,4†, Shu Zheng1,4† 1Cancer Institute (Key Laboratory of Cancer Prevention and Intervention, China National Ministry of Education), The Second Affiliated Hospital, Zhejiang University School of Medicine, Hangzhou, Zhejiang 310009, China 2Key Laboratory of Reproductive and Genetics, Ministry of Education, Women’s Hospital, Zhejiang University, Hangzhou, Zhejiang 310006, China 3Department of Abdominal Oncology, West China Hospital, Sichuan University, Chengdu, Sichuan 610041, China 4Research Center for Air Pollution and Health, School of Medicine, Zhejiang University, Hangzhou, Zhejiang 310009, China *These authors contributed equally to this work †Correspondence: Wangxiong Hu Tel: +86-571-87784606 Mailing address: 88 Jiefang Rd, Hangzhou, China 310009 E-mail: [email protected] Weiting Ge Tel: +86-571-87784606 Mailing address: 88 Jiefang Rd, Hangzhou, China 310009 E-mail: [email protected] Suzhan Zhang Tel: +86-571-87784501 Mailing address: 88 Jiefang Rd, Hangzhou, China 310009 1 Downloaded from mcr.aacrjournals.org on September 23, 2021. © 2017 American Association for Cancer Research. Author Manuscript Published OnlineFirst on November 29, 2017; DOI: 10.1158/1541-7786.MCR-17-0483 Author manuscripts have been peer reviewed and accepted for publication but have not yet been edited. E-mail: [email protected] Shu Zheng Tel: +86-571-87784501 Fax: +86-571-87214404 Mailing address: 88 Jiefang Rd, Hangzhou, China 310009 E-mail: [email protected] Conflicts of interest The authors declare that they have no competing financial interests. -
Towards an Integrated View of Wnt Signaling in Development Renée Van Amerongen and Roel Nusse*
HYPOTHESIS 3205 Development 136, 3205-3214 (2009) doi:10.1242/dev.033910 Towards an integrated view of Wnt signaling in development Renée van Amerongen and Roel Nusse* Wnt signaling is crucial for embryonic development in all animal Notably, components at virtually every level of the Wnt signal species studied to date. The interaction between Wnt proteins transduction cascade have been shown to affect both β-catenin- and cell surface receptors can result in a variety of intracellular dependent and -independent responses, depending on the cellular responses. A key remaining question is how these specific context. As we discuss below, this holds true for the Wnt proteins responses take shape in the context of a complex, multicellular themselves, as well as for their receptors and some intracellular organism. Recent studies suggest that we have to revise some of messengers. Rather than concluding that these proteins are shared our most basic ideas about Wnt signal transduction. Rather than between pathways, we instead propose that it is the total net thinking about Wnt signaling in terms of distinct, linear, cellular balance of signals that ultimately determines the response of the signaling pathways, we propose a novel view that considers the receiving cell. In the context of an intact and developing integration of multiple, often simultaneous, inputs at the level organism, cells receive multiple, dynamic, often simultaneous and of both Wnt-receptor binding and the downstream, sometimes even conflicting inputs, all of which are integrated to intracellular response. elicit the appropriate cell behavior in response. As such, the different signaling pathways might thus be more intimately Introduction intertwined than previously envisioned.