Unusual Structural Features Revealed by the Solution NMR Structure of the NLRC5 Caspase Recruitment Domain
Total Page:16
File Type:pdf, Size:1020Kb
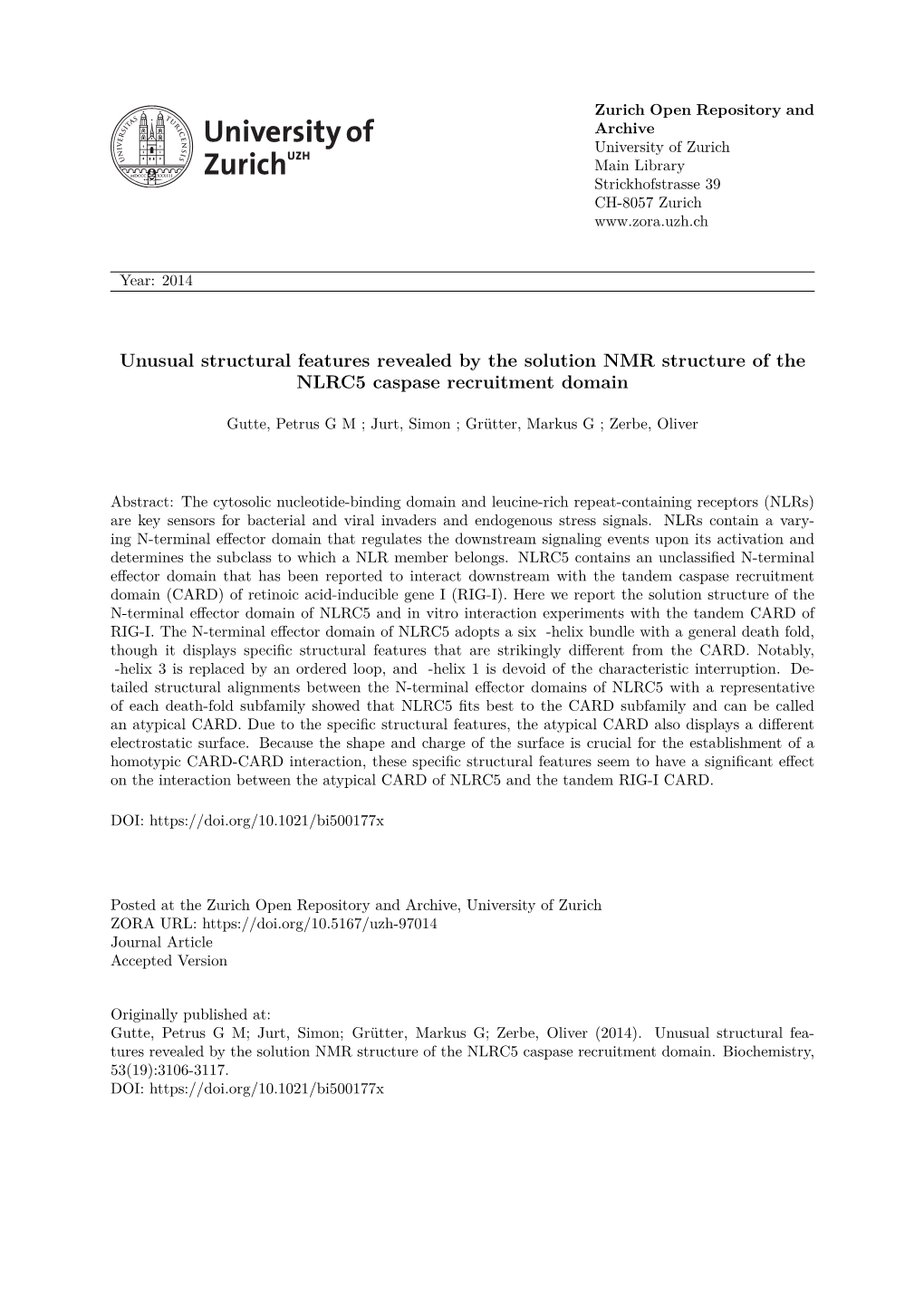
Load more
Recommended publications
-
Dissertation Philip Böhler
Three Tales of Death: New Pathways in the Induction, Inhibition and Execution of Apoptosis Inaugural-Dissertation zur Erlangung des Doktorgrades der Mathematisch-Naturwissenschaftlichen Fakultät der Heinrich-Heine-Universität Düsseldorf vorgelegt von Philip Böhler aus Bonn Düsseldorf, Juni 2019 aus dem Institut für Molekulare Medizin I der Heinrich-Heine-Universität Düsseldorf Gedruckt mit der Genehmigung der Mathematisch-Naturwissenschaftlichen Fakultät der Heinrich-Heine-Universität Düsseldorf Berichterstatter: 1. Prof. Dr. Sebastian Wesselborg 2. Prof. Dr. Henrike Heise Tag der mündlichen Prüfung: 29. Oktober 2019 “Where the first primal cell was, there was I also. Where man is, there am I. When the last life crawls under freezing stars, there will I be.” — DEATH, in: Mort, by Terry Pratchett “Right away I found out something about biology: it was very easy to find a question that was very interesting, and that nobody knew the answer to.” — Richard Feynman, in: Surely You're Joking, Mr. Feynman! Acknowledgements (Danksagung) Acknowledgements (Danksagung) Viele Menschen haben zum Gelingen meiner Forschungsarbeit und dieser Dissertation beigetragen, und nicht alle können hier namentlich erwähnt werden. Dennoch möchte ich einige besonders hervorheben. An erster Stelle möchte ich Professor Sebastian Wesselborg danken, der diese Dissertation als Erstgutachter betreut hat und der mir die Möglichkeit gab, die dazugehörigen experimentellen Arbeiten am Institut für Molekulare Medizin durchzuführen. Er und Professor Björn Stork, dem ich für die herzliche Aufnahme in seine Arbeitsgruppe danke, legten durch die richtige Mischung aus aktiver Förderung und dem Freiraum zur Umsetzung eigener wissenschaftlicher Ideen die ideale Grundlage für die Forschungsprojekte, aus denen diese Dissertation entstand. Professorin Henrike Heise, die sich freundlicherweise zur Betreuung dieser Dissertation als Zweitgutachterin bereiterklärt hat, gilt ebenfalls mein herzlicher Dank. -
Supplementary A
Genomic Analysis of the Immune Gene Repertoire of Amphioxus Reveals Extraordinary Innate Complexity and Diversity Supplementary A Content 1 TLR system....................................................................................................................................2 2 NLR system ...................................................................................................................................4 3 LRRIG genes .................................................................................................................................5 4 Other LRR-containing models.......................................................................................................6 5 Domain combinations in amphioxus C-type lectins ......................................................................8 References.........................................................................................................................................9 Table S1. Cross-species comparison of the immune-related protein domains................................10 Table S2. Information of 927 amphioxus CTL gene models containing single CTLD domain. ....11 Table S3. Grouping of the amphioxus DFD gene models based on their architectures..................12 Figure S1. Two structural types of TLR. ........................................................................................13 Figure S2. Phylogenetic analysis of amphioxus P-TLRs and all vertebrate TLR families.............14 Figure S3. Phylogenetic analysis of amphioxus TLRs -
Familial Cortical Myoclonus Caused by Mutation in NOL3 by Jonathan Foster Rnsseil DISSERTATION Submitted in Partial Satisfaction
Familial Cortical Myoclonus Caused by Mutation in NOL3 by Jonathan Foster Rnsseil DISSERTATION Submitted in partial satisfaction of the requirements for the degree of DOCTOR OF PHILOSOPHY in Biomedical Sciences in the Copyright 2013 by Jonathan Foster Russell ii I dedicate this dissertation to Mom and Dad, for their adamantine love and support iii No man has earned the right to intellectual ambition until he has learned to lay his course by a star which he has never seen—to dig by the divining rod for springs which he may never reach. In saying this, I point to that which will make your study heroic. For I say to you in all sadness of conviction, that to think great thoughts you must be heroes as well as idealists. Only when you have worked alone – when you have felt around you a black gulf of solitude more isolating than that which surrounds the dying man, and in hope and in despair have trusted to your own unshaken will – then only will you have achieved. Thus only can you gain the secret isolated joy of the thinker, who knows that, a hundred years after he is dead and forgotten, men who never heard of him will be moving to the measure of his thought—the subtile rapture of a postponed power, which the world knows not because it has no external trappings, but which to his prophetic vision is more real than that which commands an army. -Oliver Wendell Holmes, Jr. iv ACKNOWLEDGMENTS I am humbled by the efforts of many, many others who were essential for this work. -
NLRP3) Inflammasome Activity Is Regulated by and Potentially Targetable Through Bruton Tyrosine Kinase
Human NACHT, LRR, and PYD domain-containing protein 3 (NLRP3) inflammasome activity is regulated by and potentially targetable through Bruton tyrosine kinase Thesis submitted as requirement to fulfill the degree „Doctor of Philosophy“ (Ph.D.) at the Faculty of Medicine Eberhard Karls University Tübingen by Xiao Liu (刘晓) from Shandong, China 2018 1 Dean: Professor Dr. I. B. Autenrieth 1. Reviewer: Professor A. Weber 2. Reviewer: Professor S. Beer-Hammer 2 Content Content Figures ..................................................................................................................................................... iv Tables ....................................................................................................................................................... vi Abbreviations ........................................................................................................................................ vii 1 Introduction ....................................................................................................................................... 1 1.1 The human immune system .................................................................................................... 1 1.1.1 Innate immune response ................................................................................................................... 1 1.1.2 Adaptive immune response ............................................................................................................. 2 1.2 Inflammasomes are a group of -
The Conservation and Uniqueness of the Caspase Family in the Basal
Xu et al. BMC Biology 2011, 9:60 http://www.biomedcentral.com/1741-7007/9/60 RESEARCHARTICLE Open Access The conservation and uniqueness of the caspase family in the basal chordate, amphioxus Liqun Xu†, Shaochun Yuan†, Jun Li, Jie Ruan, Shengfeng Huang, Manyi Yang, Huiqing Huang, Shangwu Chen, Zhenghua Ren and Anlong Xu* Abstract Background: The caspase family, which plays a central role in apoptosis in metazoans, has undergone an expansion in amphioxus, increasing to 45 members through domain recombination and shuffling. Results: In order to shed light on the conservation and uniqueness of this family in amphioxus, we cloned three representative caspase genes, designated as bbtCaspase-8, bbtCaspase-1/2 and bbtCaspase3-like, from the amphioxus Branchiostoma belcheri tsingtauense. We found that bbtCaspase-8 with conserved protein architecture is involved in the Fas-associated death domain-Caspase-8 mediated pro-apoptotic extrinsic pathway, while bbtCaspase3-like may mediate a nuclear apoptotic pathway in amphioxus. Also, bbtCaspase-1/2 can co-localize with bbtFADD2 in the nucleus, and be recruited to the cytoplasm by amphioxus apoptosis associated speck-like proteins containing a caspase recruitment domain, indicating that bbtCaspase-1/2 may serve as a switch between apoptosis and caspase-dependent innate immune response in invertebrates. Finally, amphioxus extrinsic apoptotic pathway related caspases played important roles in early embryogenesis. Conclusions: Our study not only demonstrates the conservation of bbtCaspase-8 in apoptosis, but also reveals the unique features of several amphioxus caspases with novel domain architectures arose some 500 million years ago. Background intrinsic pathway is triggered by death stimuli generated Programmed cell death is a gene-guided process for the within the cell, such as DNA damage, leading to the elimination of unnecessary or harmful cells in which the release of mitochondrial cytochrome c, which associates cysteine proteases caspases are core elements [1-3]. -
Assembly and Regulation of the Death Effector Domain Complexes
Citation: Cell Death and Disease (2015) 6, e1866; doi:10.1038/cddis.2015.213 OPEN & 2015 Macmillan Publishers Limited All rights reserved 2041-4889/15 www.nature.com/cddis Review DED or alive: assembly and regulation of the death effector domain complexes JS Riley1, A Malik1, C Holohan1 and DB Longley*,1 Death effector domains (DEDs) are protein–protein interaction domains initially identified in proteins such as FADD, FLIP and caspase-8 involved in regulating apoptosis. Subsequently, these proteins have been shown to have important roles in regulating other forms of cell death, including necroptosis, and in regulating other important cellular processes, including autophagy and inflammation. Moreover, these proteins also have prominent roles in innate and adaptive immunity and during embryonic development. In this article, we review the various roles of DED-containing proteins and discuss recent developments in our understanding of DED complex formation and regulation. We also briefly discuss opportunities to therapeutically target DED complex formation in diseases such as cancer. Cell Death and Disease (2015) 6, e1866; doi:10.1038/cddis.2015.213; published online 27 August 2015 Facts Are DED-containing proteins potential therapeutic targets for inflammatory diseases? FADD, FLIP, procaspase-8 and procaspase-10 all contain death effector domains (DEDs). Cell death is critical for maintaining homeostasis in multi- The DED is a conserved protein sub-domain that mediates cellular organisms; too much can result in pathologies such as important protein–protein interactions. neurodegeneration, whereas too little can lead to the DED-containing proteins form a variety of complexes that accumulation of malignant cancerous cells. -
The Death Domain Superfamily in Intracellular Signaling of Apoptosis and Inflammation
ANRV306-IY25-19 ARI 11 February 2007 12:51 The Death Domain Superfamily in Intracellular Signaling of Apoptosis and Inflammation Hyun Ho Park,1 Yu-Chih Lo,1 Su-Chang Lin,1 Liwei Wang,1 Jin Kuk Yang,1,2 and Hao Wu1 1Department of Biochemistry, Weill Medical College and Graduate School of Medical Sciences of Cornell University, New York, New York 10021; email: [email protected] 2Department of Chemistry, Soongsil University, Seoul 156-743, Korea Annu. Rev. Immunol. 2007. 25:561–86 Key Words First published online as a Review in Advance on death domain (DD), death effector domain (DED), tandem DED, January 2, 2007 caspase recruitment domain (CARD), pyrin domain (PYD), crystal The Annual Review of Immunology is online at structure, NMR structure immunol.annualreviews.org This article’s doi: Abstract 10.1146/annurev.immunol.25.022106.141656 The death domain (DD) superfamily comprising the death domain Copyright c 2007 by Annual Reviews. (DD) subfamily, the death effector domain (DED) subfamily, the Annu. Rev. Immunol. 2007.25:561-586. Downloaded from arjournals.annualreviews.org by CORNELL UNIVERSITY MEDICAL COLLEGE on 03/29/07. For personal use only. All rights reserved caspase recruitment domain (CARD) subfamily, and the pyrin do- 0732-0582/07/0423-0561$20.00 main (PYD) subfamily is one of the largest domain superfamilies. By mediating homotypic interactions within each domain subfam- ily, these proteins play important roles in the assembly and activation of apoptotic and inflammatory complexes. In this chapter, we review the molecular complexes assembled by these proteins, the structural and biochemical features of these domains, and the molecular in- teractions mediated by them. -
Osu1211550109.Pdf (2.72
ROLE OF IKAPPABZETA AND PYRIN AS MODULATORS OF MACROPHAGE INNATE IMMUNE FUNCTION DISSERTATION Presented in Partial Fulfillment of the Requirements for the Degree Doctor of Philosophy in the Graduate School of The Ohio State University By Sudarshan Seshadri, M.S * * * * * The Ohio State University 2008 Dissertation Committee: Dr. Mark Wewers, Advisor Approved by Dr. Susheela Tridandapani Dr. Scott Walsh Dr. Daren Knoell Advisor Biophysics Graduate Program ABSTRACT Innate immunity is the first line of defense against the pathogens mounted by the host. The host response mediated by innate immunity is quick and takes place within the first few hours after the pathogen invasion. Proper functioning of innate immunity is required for mounting the adaptive immune response. All lower order organisms, animals and plants rely on innate immunity as their prime mode of defense. However, studies on innate immunity have been very limited so far. Innate immune responses are initiated by three main receptors, toll like receptors, nucleotide oligomerization domain-like receptors and RIG-like receptors. These receptors get activated upon pathogen recognition and turn on several proinflammatory pathways. The present study concentrated on two proinflammatory pathways, the signalosome and the inflammasome pathway. The signalosome pathway leads to the production of the pro-inflammatory cytokines that are involved in host defense and also regulates the expression of proteins that are involved in host cell survival. IL-1β is one such cytokine dependent on signalosome pathway for its production. However, the produced IL-1β lacks biological activity and it needs to be processed to mature biologically active IL-1β. This process of converting the proIL-1β to mature form requires a cysteine protease known as caspase-1. -
Genes Involved and Proteins
Atlas of Genetics and Cytogenetics in Oncology and Haematology OPEN ACCESS JOURNAL AT INIST-CNRS Scope The Atlas of Genetics and Cytogenetics in Oncology and Haematology is a peer reviewed on-line journal in open access, devoted to genes, cytogenetics, and clinical entities in cancer, and cancer-prone diseases. It presents structured review articles ("cards") on genes, leukaemias, solid tumours, cancer-prone diseases, more traditional review articles on these and also on surrounding topics ("deep insights"), case reports in hematology, and educational items in the various related topics for students in Medicine and in Sciences. Editorial correspondance Jean-Loup Huret Genetics, Department of Medical Information, University Hospital F-86021 Poitiers, France tel +33 5 49 44 45 46 or +33 5 49 45 47 67 [email protected] or [email protected] Staff Mohammad Ahmad, Mélanie Arsaban, Houa Delabrousse, Marie-Christine Jacquemot-Perbal, Maureen Labarussias, Vanessa Le Berre, Anne Malo, Catherine Morel-Pair, Laurent Rassinoux, Sylvie Yau Chun Wan - Senon, Alain Zasadzinski. Philippe Dessen is the Database Director, and Alain Bernheim the Chairman of the on-line version (Gustave Roussy Institute – Villejuif – France). The Atlas of Genetics and Cytogenetics in Oncology and Haematology (ISSN 1768-3262) is published 12 times a year by ARMGHM, a non profit organisation, and by the INstitute for Scientific and Technical Information of the French National Center for Scientific Research (INIST-CNRS) since 2008. The Atlas is hosted by INIST-CNRS (http://www.inist.fr) http://AtlasGeneticsOncology.org © ATLAS - ISSN 1768-3262 The PDF version of the Atlas of Genetics and Cytogenetics in Oncology and Haematology is a reissue of the original articles published in collaboration with the Institute for Scientific and Technical Information (INstitut de l’Information Scientifique et Technique - INIST) of the French National Center for Scientific Research (CNRS) on its electronic publishing platform I-Revues. -
And Sepsis the Host Response To
The Inflammatory Caspases: Key Players in the Host Response to Pathogenic Invasion and Sepsis This information is current as Amal Nadiri, Melissa K. Wolinski and Maya Saleh of September 29, 2021. J Immunol 2006; 177:4239-4245; ; doi: 10.4049/jimmunol.177.7.4239 http://www.jimmunol.org/content/177/7/4239 Downloaded from References This article cites 91 articles, 31 of which you can access for free at: http://www.jimmunol.org/content/177/7/4239.full#ref-list-1 Why The JI? Submit online. http://www.jimmunol.org/ • Rapid Reviews! 30 days* from submission to initial decision • No Triage! Every submission reviewed by practicing scientists • Fast Publication! 4 weeks from acceptance to publication *average by guest on September 29, 2021 Subscription Information about subscribing to The Journal of Immunology is online at: http://jimmunol.org/subscription Permissions Submit copyright permission requests at: http://www.aai.org/About/Publications/JI/copyright.html Email Alerts Receive free email-alerts when new articles cite this article. Sign up at: http://jimmunol.org/alerts The Journal of Immunology is published twice each month by The American Association of Immunologists, Inc., 1451 Rockville Pike, Suite 650, Rockville, MD 20852 Copyright © 2006 by The American Association of Immunologists All rights reserved. Print ISSN: 0022-1767 Online ISSN: 1550-6606. THE JOURNAL OF IMMUNOLOGY BRIEF REVIEWS The Inflammatory Caspases: Key Players in the Host Response to Pathogenic Invasion and Sepsis Amal Nadiri,1* Melissa K. Wolinski,1† and Maya Saleh2* Caspases are cysteinyl-aspartate-specific proteinases lar partners and may therefore share common mechanisms of known for their role in apoptosis (cell death or apoptotic activation. -
Mechanisms Involved in the Lack of Immune Reconstitution During Antiretroviral Therapy in HIV- Infected Individuals
UNIVERSITÀ DEGLI STUDI DI MILANO DOTTORATO IN MEDICINA MOLECOLARE E TRASLAZIONALE CICLO XXIX Anno Accademico 2015/2016 TESI DI DOTTORATO DI RICERCA MED/04 – Patologia Generale Mechanisms Involved in the Lack of Immune Reconstitution during Antiretroviral Therapy in HIV- Infected Individuals Dottorando: Michela MASETTI Matricola N°R10790 TUTORE : Ch.mo Prof. Mario Salvatore CLERICI CO-TUTORE: Prof.ssa Daria TRABATTONI DIRETTORE DEL DOTTORATO: Ch.mo Prof. Mario Salvatore CLERICI “The important thing is to not stop questioning. Curiosity has its own reason for existence. One cannot help but be in awe when he contemplates the mysteries of eternity, of life, of the marvelous structure of reality. It is enough if one tries merely to comprehend a little of this mystery each day.” —"Old Man's Advice to Youth: 'Never Lose a Holy Curiosity.'" LIFE Magazine (2 May 1955) p. 64” ― Albert Einstein Abstract Introduction: HIV infection is characterized by CD4+ T cell immunodeficiency and chronic inflammation. Antiretroviral therapy (ART) leads to the recovery of CD4+ T cells by suppressing viral replication. However 15-30% of HIV-infected ART-treated patients fail to restore CD4+ T cells despite full viral suppression and they are known as “Immunological Non-Responders (INR)”. INRs are characterized by higher risk of AIDS progression and non-AIDS-related morbidity compared to HIV- infected ART-treated immunological responder (IR) patients. Several mechanisms have been involved in immune failure, however none of them provides a full explanation for the lack of immune reconstitution observed in INRs. Inflammasomes are multimeric protein platforms involved in the regulation of inflammatory responses, Th17 activity and in a high inflammatory form of programmed cell-death called “pyroptosis”. -
DED Or Alive: Assembly and Regulation of the Death Effector Domain Complexes
DED or alive: assembly and regulation of the death effector domain complexes. Riley, JS., Malik, A., Holohan, C., & Longley, DB. (2015). DED or alive: assembly and regulation of the death effector domain complexes. Cell, Death & Disease, 6(e1866), [e1866]. https://doi.org/10.1038/cddis.2015.213 Published in: Cell, Death & Disease Document Version: Publisher's PDF, also known as Version of record Queen's University Belfast - Research Portal: Link to publication record in Queen's University Belfast Research Portal Publisher rights © 2015, The Authors Cell Death and Disease is an open-access journal published by Nature Publishing Group. This work is licensed under a Creative Commons Attribution 4.0 International License. The images or other third party material in this article are included in the article’s Creative Commons license, unless indicated otherwise in the credit line; if the material is not included under the Creative Commons license, users will need to obtain permission from the license holder to reproduce the material. To view a copy of this license, visit http://creativecommons.org/licenses/by/4.0/. General rights Copyright for the publications made accessible via the Queen's University Belfast Research Portal is retained by the author(s) and / or other copyright owners and it is a condition of accessing these publications that users recognise and abide by the legal requirements associated with these rights. Take down policy The Research Portal is Queen's institutional repository that provides access to Queen's research output. Every effort has been made to ensure that content in the Research Portal does not infringe any person's rights, or applicable UK laws.