Energy and Exergy Analysis of a Novel Turbo-Compounding System For
Total Page:16
File Type:pdf, Size:1020Kb
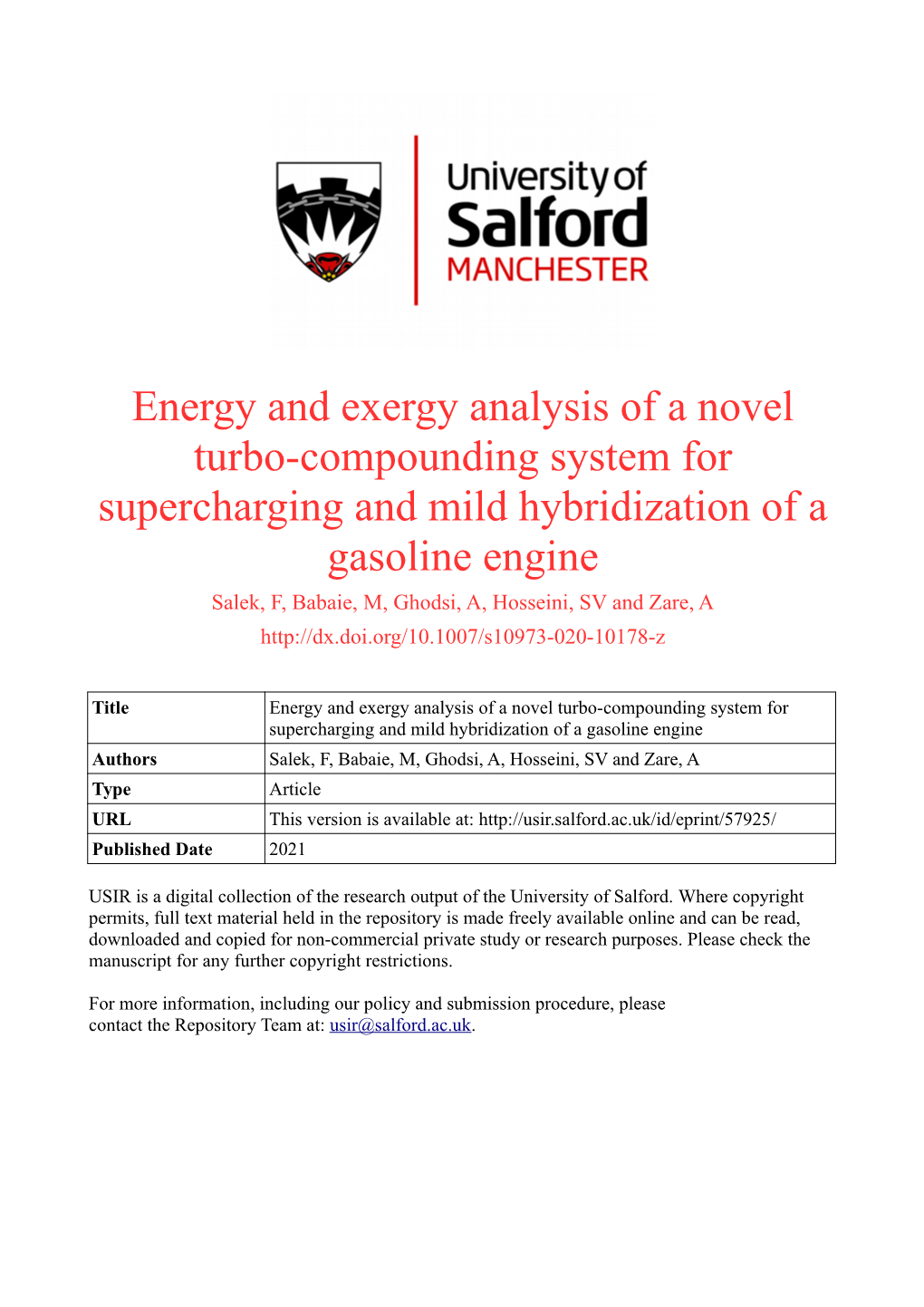
Load more
Recommended publications
-
Geothermal Power Plants and Novel Exhaust Heat Recovery Systems
Maximizing power output of heat engines through design optimization: Geothermal power plants and novel exhaust heat recovery systems Thèse Noémie Chagnon-Lessard Doctorat en génie mécanique Philosophiæ doctor (Ph. D.) Québec, Canada © Noémie Chagnon-Lessard, 2020 Maximizing power output of heat engines through design optimization: Geothermal power plants and novel exhaust heat recovery systems Thèse Noémie Chagnon-Lessard Sous la direction de : François Mathieu-Potvin, directeur de recherche Louis Gosselin, codirecteur de recherche Résumé Le design de machines thermiques menant à une puissance maximale dépend souvent des températures de la source chaude et de la source froide. C’est pourquoi dégager des lignes directrices à partir des designs optimaux de ces machines selon diverses températures d’opération peut faciliter leur conception. Une telle étude est proposée par cette thèse pour deux types de systèmes thermiques. En premier lieu, le cycle de Rankine organique (ORC) est un cycle thermodynamique de puissance utilisé entre autres dans les centrales géothermiques exploitant des réservoirs à basse température. Depuis quelques années, ce type de centrales suscite un vif intérêt à travers le monde, étant un des modes de production de puissance parmi les plus respectueux de l’environnement. Il s’agit de pomper un géofluide du sol pour transférer sa chaleur à un fluide de travail qui opère en cycle fermé, et de le réinjecter ensuite dans le bassin géologique. Les chercheurs tentent actuellement de mieux caractériser le potentiel géothermique de divers environnements géologiques. Le sous-sol du Québec est relativement froid, alors des études essaient de déterminer s’il serait possible d’y exploiter de manière rentable des centrales géothermiques. -
Inverted Brayton Cycles for Exhaust Gas Energy Recovery
University of Bath PHD Inverted Brayton Cycles for Exhaust Gas Energy Recovery Chen, Zhihang Award date: 2019 Link to publication Alternative formats If you require this document in an alternative format, please contact: [email protected] General rights Copyright and moral rights for the publications made accessible in the public portal are retained by the authors and/or other copyright owners and it is a condition of accessing publications that users recognise and abide by the legal requirements associated with these rights. • Users may download and print one copy of any publication from the public portal for the purpose of private study or research. • You may not further distribute the material or use it for any profit-making activity or commercial gain • You may freely distribute the URL identifying the publication in the public portal ? Take down policy If you believe that this document breaches copyright please contact us providing details, and we will remove access to the work immediately and investigate your claim. Download date: 08. Oct. 2021 Inverted Brayton Cycles for Exhaust Gas Energy Recovery Zhihang Chen A thesis submitted for the degree of Doctor of Philosophy University of Bath Department of Mechanical Engineering April 2019 COPYRIGHT Attention is drawn to the fact that the copyright of this thesis rests with its author and copyright of any previously published materials included may rest with third parties. A copy of this thesis has been supplied on condition that anyone who consults it understands that they must not copy it or use material from it except as licenced, permitted by law or with the consent of the author or other copyright owners, as applicable. -
Download Full Text Article (PDF)
INTERNATIONAL JOURNAL OF ENERGY AND ENVIRONMENT Volume 3, Issue 5, 2012 pp.715-730 Journal homepage: www.IJEE.IEEFoundation.org Exergy analysis for combined regenerative Brayton and inverse Brayton cycles Zelong Zhang, Lingen Chen, Fengrui Sun College of Naval Architecture and Power, Naval University of Engineering, Wuhan 430033, China. Abstract This paper presents the study of exergy analysis of combined regenerative Brayton and inverse Brayton cycles. The analytical formulae of exergy loss and exergy efficiency are derived. The largest exergy loss location is determined. By taking the maximum exergy efficiency as the objective, the choice of bottom cycle pressure ratio is optimized by detailed numerical examples, and the corresponding optimal exergy efficiency is obtained. The influences of various parameters on the exergy efficiency and other performances are analyzed by numerical calculations. Copyright © 2012 International Energy and Environment Foundation - All rights reserved. Keywords: Regenerative Brayton cycle; Inverse Brayton cycle; Exergy analysis; Exergy loss; Exergy efficiency; Optimization. 1. Introduction Nowadays, in order to meet the demands of energy-saving and environmental protection, people want to construct new energy and power plants which could gain better performance. Because of their high efficiency and advances in the technologies of the individual components, combined-cycle power plants have been applied widely in recent years. Steam and gas turbine combined cycles are considered the most effective power plants [1]. The thermal efficiency of these cycle types exceeded 55 percent several years ago and is now at approximately 60 percent. Also these cycle types’ application is becoming more and more common in mid and large scale power production due to their high efficiency and reliability. -
Bottoming Cycles for Electric Energy Generation: Parametric
Applied Energy 88 (2011) 1500–1509 Contents lists available at ScienceDirect Applied Energy journal homepage: www.elsevier.com/locate/apenergy Bottoming cycles for electric energy generation: Parametric investigation of available and innovative solutions for the exploitation of low and medium temperature heat sources ⇑ M. Bianchi, A. De Pascale Università di Bologna, Viale del Risorgimento 2, 40136 Bologna, Italy article info abstract Article history: Many industrial processes and conventional fossil fuel energy production systems used in small-medium Received 29 October 2009 industries, such as internal combustion engines and gas turbines, provide low or medium temperature Received in revised form 8 November 2010 (i.e., 200–500 °C) heat fluxes as a by-product, which are typically wasted in the environment. The possi- Accepted 11 November 2010 bility of exploiting this wasted heat, converting it into electric energy by means of different energy sys- Available online 28 December 2010 tems, is investigated in this article, by extending the usual range of operation of existing technologies or introducing novel concepts. In particular, among the small size bottoming cycle technologies, the identi- Keywords: fied solutions which could allow to improve the energy saving performance of an existing plant by gen- Heat recovery erating a certain amount of electric energy are: the Organic Rankine Cycle, the Stirling engine and the Bottoming cycle Inverted Brayton Cycle (IBC) Inverted Brayton Cycle; this last is an original thermodynamic concept included in the performed com- Organic Rankine Cycle (ORC) parative analysis. Stirling Moreover, this paper provides a parametric investigation of the thermodynamic performance of the dif- ferent systems; in particular, for the Inverted Brayton Cycle, the effects of the heat source characteristics and of the cycle design parameters on the achievable efficiency and specific power are shown. -
Energy Performance Optimization of Combined Brayton and Two Parallel Inverse Brayton Cycles with Regeneration Before the Inverse Cycles
View metadata, citation and similar papers at core.ac.uk brought to you by CORE provided by Elsevier - Publisher Connector Scientia Iranica B (2012) 19 (5), 1279–1287 Sharif University of Technology Scientia Iranica Transactions B: Mechanical Engineering www.sciencedirect.com Energy performance optimization of combined Brayton and two parallel inverse Brayton cycles with regeneration before the inverse cycles Z. Zhang, L. Chen ∗, F. Sun College of Naval Architecture and Power, Naval University of Engineering, Wuhan 430033, China Received 10 August 2011; revised 4 June 2012; accepted 17 July 2012 KEYWORDS Abstract This paper proposes combined regenerative Brayton and two parallel inverse Brayton cycles Regenerative Brayton cycle; with regeneration before the inverse cycles. Performance analysis and optimization for the combined cycle Two parallel inverse Brayton are performed based on the first law. The analytical formulae of thermal efficiency and specific work are cycles; derived. The performance analysis and optimization of thermal efficiency and specific work are carried The first law analysis; out by adjusting the compressor pressure ratios of the bottom cycles. The influences of the effectiveness Thermal efficiency; of the regenerator and other parameters on the optimal thermal efficiency and the optimal specific work Specific work; are analyzed by numerical examples. It is found that the combined regenerative cycle can obtain higher Performance optimization. thermal efficiency than that of the base cycle but with smaller specific work. It is revealed that if the effectiveness of regenerator equals to 0.9, the combined cycles will attain an optimal thermal efficiency of 51.2%. ' 2012 Sharif University of Technology. Production and hosting by Elsevier B.V. -
Experimental Investigation of an Inverted Brayton Cycle for Exhaust Gas Energy Recovery
Proceedings of ASME Turbo Expo 2018 Turbomachinery Technical Conference and Exposition GT2018 June 11-15, 2018, Oslo, Norway GT2018-75386 Downloaded from http://asmedigitalcollection.asme.org/GT/proceedings-pdf/GT2018/51173/V008T26A003/3768225/v008t26a003-gt2018-75386.pdf by guest on 28 September 2021 EXPERIMENTAL INVESTIGATION OF AN INVERTED BRAYTON CYCLE FOR EXHAUST GAS ENERGY RECOVERY Ian Kennedy Zhihang Chen University of Bath University of Bath Bath, UK Bath, UK Bob Ceen Simon Jones Colin D Copeland Axes Design Ltd HIETA Technologies Ltd University of Bath Malvern, UK Bristol, UK Bath, UK ABSTRACT the vehicle. A window of opportunity still remains for this until Exhaust gases from an internal combustion engine (ICE) alternatives such as electric vehicles become more readily contain approximately 30% of the total energy released from available and cost effective. Recovering energy from exhaust combustion of the fuel. In order to improve fuel economy and gases is one key means of improving the efficiency of ICE reduce emissions, there are a number of technologies available powered vehicles. Turbomachinery can be used to recover this to recover some of the otherwise wasted energy. The inverted energy using technologies such as the IBC, which is shown Brayton cycle (IBC) is one such technology. schematically on Fig. 1. The purpose of the study is to conduct a parametric experimental investigation of the IBC. Hot air from a Coolant Heat turbocharger test facility is used. The system is sized to operate exchanger using the exhaust gases produced by a 2 litre turbocharged engine at motorway cruise conditions. A number of parameters Electric are investigated that impact the performance of the system such C T as turbine inlet temperature, system pressure drop and machine compressor inlet temperature. -
Thermodynamic Cycles for Low- and High-Temperature Waste Heat Recovery from Heavy-Duty Engines
THESIS FOR THE DEGREE OF DOCTOR OF PHILOSOPHY IN THERMO AND FLUID DYNAMICS Thermodynamic Cycles for Low- and High-Temperature Waste Heat Recovery from Heavy-Duty Engines An Experimental and Simulation Study JELMER RIJPKEMA Department of Mechanics and Maritime Sciences CHALMERS UNIVERSITY OF TECHNOLOGY Gothenburg, Sweden 2021 Thermodynamic Cycles for Low- and High-Temperature Waste Heat Recovery from Heavy-Duty Engines An Experimental and Simulation Study JELMER RIJPKEMA ISBN 978-91-7905-486-1 © JELMER RIJPKEMA, 2021 Doktorsavhandlingar vid Chalmers tekniska högskola Ny serie nr. 4953 ISSN 0346-718X Department of Mechanics and Maritime Sciences Chalmers University of Technology SE-412 96 Gothenburg Sweden Telephone: +46 (0)31-772 1000 Chalmers Reproservice Gothenburg, Sweden 2021 Thermodynamic Cycles for Low- and High-Temperature Waste Heat Recovery from Heavy-Duty Engines An Experimental and Simulation Study Thesis for the degree of Doctor of Philosophy in Thermo and Fluid Dynamics JELMER RIJPKEMA Department of Mechanics and Maritime Sciences Chalmers University of Technology Abstract To reduce the environmental impact of heavy-duty vehicles, it is critical to reduce their CO2 emissions by improving the engine efficiency. A promising way to do this is by extracting waste heat from the engine during operation and converting it into useful work. This thesis presents a comprehensive evaluation of the performance of thermodynamic cycles for waste heat recovery from heavy-duty engines. First, by identifying the com- bination(s) of heat source, -
Thermodynamic Assessment of Integrated Heat Recovery System Combining Exhaust-Gas Heat and Cold Energy for LNG Regasification Process in FSRU Vessel
Thermodynamic assessment of integrated heat recovery system combining exhaust-gas heat and cold energy for LNG regasification process in FSRU vessel The MIT Faculty has made this article openly available. Please share how this access benefits you. Your story matters. Citation Lee, Sangick, and Byung Chul Choi. “Thermodynamic Assessment of Integrated Heat Recovery System Combining Exhaust-Gas Heat and Cold Energy for LNG Regasification Process in FSRU Vessel.” J Mech Sci Technol 30, no. 3 (March 2016): 1389–1398. As Published http://dx.doi.org/10.1007/s12206-016-0246-y Publisher Korean Society of Mechanical Engineers Version Author's final manuscript Citable link http://hdl.handle.net/1721.1/104007 Terms of Use Article is made available in accordance with the publisher's policy and may be subject to US copyright law. Please refer to the publisher's site for terms of use. Journal of Mechanical Science and Technology 30 (3) (2016) 1389~1398 www.springerlink.com/content/1738-494x(Print)/1976-3824(Online) DOI 10.1007/s12206-016-0246-y Thermodynamic assessment of integrated heat recovery system combining exhaust-gas heat and cold energy for LNG regasification process in FSRU vessel† Sangick Lee1 and Byung Chul Choi1,2,* 1R&D Center, Korean Register of Shipping, Busan 618-814, Korea 2Department of Mechanical Engineering, Massachusetts Institute of Technology, MA 02139, USA (Manuscript Received May 12, 2015; Revised October 1, 2015; Accepted November 3, 2015) ---------------------------------------------------------------------------------------------------------------------------------------------------------------------------------------------------------------------------------------------------------------------------------------------- Abstract A thermodynamic assessment of an integrated heat recovery system, which simultaneously recovers both the cold energy of LNG re- leased into seawater and the exhaust gas heat of diesel generator released into ambient air during the regasification process in a LNG- FSRU vessel, has been carried out. -
Alternative Formats If You Require This Document in an Alternative Format, Please Contact: [email protected]
Citation for published version: Chagnon-Lessard, N, Copeland, C, Mathieu-Potvin, F & Gosselin, L 2020, 'Maximizing specific work output extracted from engine exhaust with novel inverted Brayton cycles over a large range of operating conditions', Energy. https://doi.org/10.1016/j.energy.2019.116350 DOI: 10.1016/j.energy.2019.116350 Publication date: 2020 Document Version Peer reviewed version Link to publication Publisher Rights CC BY-NC-ND University of Bath Alternative formats If you require this document in an alternative format, please contact: [email protected] General rights Copyright and moral rights for the publications made accessible in the public portal are retained by the authors and/or other copyright owners and it is a condition of accessing publications that users recognise and abide by the legal requirements associated with these rights. Take down policy If you believe that this document breaches copyright please contact us providing details, and we will remove access to the work immediately and investigate your claim. Download date: 29. Sep. 2021 MAXIMIZING SPECIFIC WORK OUTPUT EXTRACTED FROM ENGINE EXHAUST WITH NOVEL INVERTED BRAYTON CYCLES OVER A LARGE RANGE OF OPERATING CONDITIONS Noémie Chagnon-Lessard1, Colin Copeland2, François Mathieu-Potvin*1, Louis Gosselin1 (bold font weight for family name) 1 Department of Mechanical Engineering, 1065, Avenue de la Médecine, Université Laval, Quebec City, Quebec, Canada, G1V 0A6 2 Powertrain and Vehicle Research Centre, University of Bath, Claverton Down, Bath, BA2 7AY, United Kingdom Abstract The thermal energy contained in internal combustion engine exhaust gases can be converted into mechanical energy by using an Inverted Brayton Cycle (IBC). -
Energy Conversion with Heat Engines
Chapter IX ENERGY CONVERSION WITH HEAT ENGINES Chapter IX.— ENERGY CONVERSION WITH HEAT ENGINES Page Page Background . 329. Noise . ......................388 Thermodynamics. .. 330 WaterUse in SteamCycles . .............389 Cogeneration. .......................332 Sodium and Potassium Vapor . ...........389 The RankineCycle. ....................333 Cycle Performance . ..................336 Turbine Efficiency . ..............338 PerformanceWith Heat Recovery. ...340 PerformanceWith Backup PowerSources343 LIST OF TABLES State of the Art. ....................345 Table System Costs... ....................352 No. Page Costs of Organic RankineSystems. .. ...354 I. Organic Power-CycleWorking Fluids Used in SystemTesting or in The BraytonCycle. ....................354 Development . ...................339 System Efficiency. ..................360 2. Efficiency of Steam Rankine Devices OperationWith Fossil Backup. .........365 Operated From Storage at Reduced System Costs... ....................365 Temperature . .. ...................345 TheStirlingand Ericsson Cycles.. .......366 3. Ratio of EnergyRequired for Boiling Priniciples of Operation . ..............366 to Total EnergySenttoTurbine . .........345 Design Alternatives. ..............368 4. Small Steam RankineTurbines . .........346 Performance . ...............376 5. Design and Performance Parameters of PerformanceWithWaste Heat. .........378 Current and Projected RankineAutomotive SystemCosts. ....................379 PowerSystems . ......................347 State of the Art. ....................381 -
Comparative Analysis of Effeciency of Waste Heat Conversioninlow-Temperature Brayton Cycle
Chemical and Process Engineering 2018, 39 (1), 103–112 DOI: 10.24425/119102 COMPARATIVE ANALYSIS OF EFFECIENCY OF WASTE HEAT CONVERSION IN LOW-TEMPERATURE BRAYTON CYCLE Aleksandra Borsukiewicz∗, Piotr Stawicki 1 West Pomeranian University of Technology, Szczecin, ORC Power Plants Research and Development Centre, al. Piastów 17, 70-310 Szczecin, Poland The paper discusses the feasibility, effectiveness and validity of a gas turbine power plant, operated according to the Brayton comparative cycle in order to develop low-potential waste heat (160◦C) and convert it into electricity. Fourteen working fluids, mainly with organic origin have been examined. It can be concluded that low molecular weight working fluids allow to obtain higher power efficiency of Brayton cycle only if conversions without taking into account internal losses are considered. For the cycle that takes into account the compression conversion efficiency in the compressor and expansion in the gas turbine, the highest efficiency was obtained for the perfluoropentane working medium and other substances with relatively high molecular weight values. However, even for the cycle using internal heat recovery, the thermal efficiency of the Brayton cycle did not exceed 7%. Keywords: Brayton cycle, working fluid, low temperature source, low temperature power plant 1. INTRODUCTION The new energy policy of Poland by 2030 is aimed at improving energy efficiency, increasing energy security, developing renewable energy sources, including biofuels, developing competitive fuel and energy markets and reducing the impact of the generation of useful forms of energy on the environment. The basic directions of this policy correspond thematically with the main objectives of EU energy policy 3 × 20%.