Occurrence and Origin of Ring Schlieren in the South
Total Page:16
File Type:pdf, Size:1020Kb
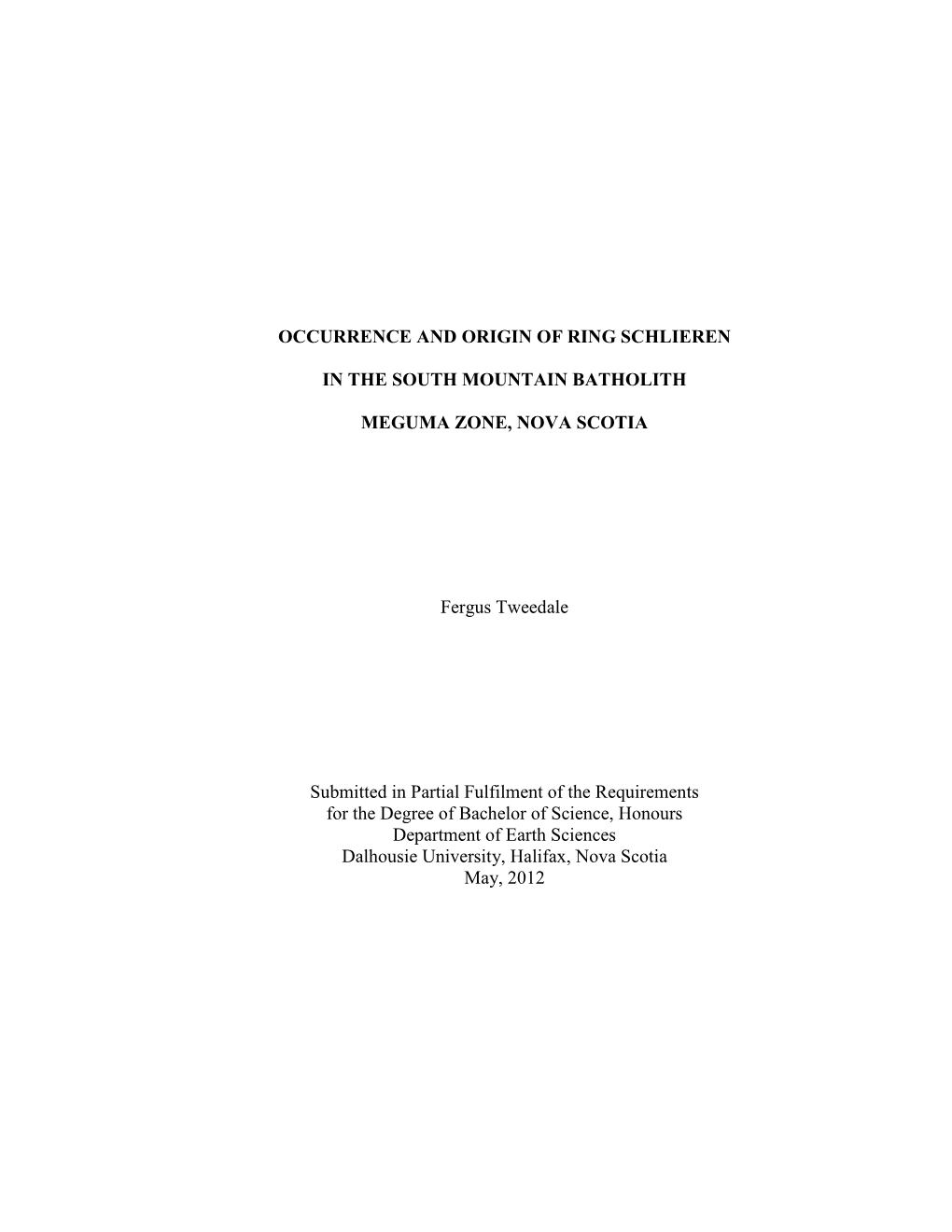
Load more
Recommended publications
-
The Acadian Fold Belt in the Meguma Terrane, Nova Scotia: Cross Sections, Fold Mechanisms, and Tectonic Implications
TECTONICS, VOL. 25, TC3007, doi:10.1029/2004TC001752, 2006 The Acadian fold belt in the Meguma Terrane, Nova Scotia: Cross sections, fold mechanisms, and tectonic implications Nicholas Culshaw1 and Sharon K. Y. Lee1 Received 6 October 2004; revised 10 January 2006; accepted 20 February 2006; published 20 May 2006. [1] Structural data and cross sections from the eastern from the Avalon Terrane (Figure 1). An Acadian fold belt part of the Devonian-Carboniferous fold belt of the affects its sedimentary component, the Cambrian–Early Meguma Terrane give insight into its structural Ordovician Meguma Group and latest Ordovician-Devonian development and crustal tectonics. The sandy White Rock, Kentville, and Torbrook formations. The fold Goldenville Formation, the active competent member belt is notable for its apparent simplicity: the locally during buckling, formed a multilayer several kilometers periclinal folds have mostly upright axial surfaces and horizontal hinges, with kilometric spacing between axial thick that lay beneath the denser, softer muds and silts of traces that are up to several tens of kilometers in length. the Halifax Formation. Cross sections reveal 2 orders of Given the external position of the Meguma Group relative folds: 11–18 km wavelength folds are interpreted as to the Appalachian orogen, the simple geometry of the resulting from buckling under the influence of gravity; Acadian fold belt is of interest, for, although resembling 4–6 km wavelength folds reflect the thickness of the an external foreland fold and thrust belt, thrusts are absent at multilayer with buckle shortening in the range 32– the present level of exposure. Also, the presence or absence 44%. -
Assessment of Geothermal Resources in Onshore Nova Scotia
FINAL REPORT Assessment of geothermal resources in onshore Nova Scotia Setting the stage, demonstrating value, and identifying next steps Félix-Antoine Comeau1 Stephan Séjourné 2 Jasmin Raymond1 1Institut national de la recherche scientifique (INRS), Centre Eau Terre Environnement 2Enki GeoSolutions inc. Rapport de recherche R2002 Prepared for the account of: Offshore Energy Research Association (OERA) December 1st, 2020 © INRS, Centre - Eau Terre Environnement, 2020 Tous droits réservés ISBN : 978-2-89146-939-5 (version électronique) Dépôt légal - Bibliothèque et Archives nationales du Québec, 2020 Dépôt légal - Bibliothèque et Archives Canada, 2020 Table of Contents ACKNOWLEDGEMENTS.................................................................................................................. 13 FOREWORD ......................................................................................................................................... 15 EXECUTIVE SUMMARY ................................................................................................................... 17 1. OVERVIEW OF THE GEOTHERMAL RESOURCE TYPES ............................................... 21 1.1 Geothermal systems ............................................................................................................... 21 1.1.1 Magmatic .......................................................................................................................... 25 1.1.2 Sedimentary basins .......................................................................................................... -
Possible Evidence for a Protracted Gold-Forming System
Further Re-Os Arsenopyrite Geochronology from Selected Meguma Au Deposits, Meguma Terrane, Nova Scotia: Possible Evidence for a Protracted Gold-forming System by Lin Chen A thesis submitted in partial fulfillment of the requirements for the degree of Master of Science Department of Earth and Atmospheric Sciences University of Alberta ©Lin Chen, 2015 Abstract The Meguma terrane, Nova Scotia, is dominated by two rock types; regionally deformed and metamorphosed Cambro-Ordovician metasedimentary rocks and ca. 380-370 Ma meta- to peraluminous granites. The metasedimentary rocks host numerous orogenic-type, vein-hosted gold deposits which occur throughout the metasandstone-dominated Goldenville Group rather than the overlying metasiltstone- and metamudstone-dominated Halifax Group. These mineralized veins are dominated by quartz-carbonate-sulfide assemblages and occupy structures consistent with emplacement during late-stage fold tightening of the regional, northeast-trending, upright folds that formed during the Acadian orogenic event at ca. 410-400 Ma. From previous work, vein formation, hence gold emplacement, spanned 30-40 Ma, as constrained from field observations and radiometric dating. The former indicates veins post-date cleavage formation given that cleaved wall-rock fragments occur in some veins, and that rarely; veins post-date hornfels related to 380 Ma granites. Existing absolute age dating indicates two events at 408 Ma (Re-Os Arsenopyrite; 40Ar/39Ar whole rock) and 380-362 Ma (Re-Os Arsenopyrite; 40Ar/39Ar Muscovite, Biotite, whole rock). Here we report new Re-Os geochronological data generated from arsenopyrite in gold-bearing veins for two deposits sampled, all of which lie in the same stratigraphic-structural position in the lower part of the Goldenville Group. -
Variscan Accretionary Complex of Northwest Iberia: Terrane Correlation and Succession of Tectonothermal Events
View metadata, citation and similar papers at core.ac.uk brought to you by CORE provided by EPrints Complutense Variscan accretionary complex of northwest Iberia: Terrane correlation and succession of tectonothermal events Jose R. Martlnez Catalan Departamento de Geologia, Unlversldad de Salamanca, 37008 Salamanca, Spain Ricardo Arenas Departamento de Petroiogia y Geoquimlca, Unlversldad Complutense, 28040 Madrid, Spain Florentino Diaz Garcia Departamento de Geologia, Unlversldad de OVledo, 33005 OVledo, Spain Jacobo Abati Departamento de Petroiogia y Geoquimlca, Unlversldad Complutense, 28040 Madrid, Spain ABSTRACT The allochthonous terranes of northwest Iberia can be correlated with specific pale ogeo graphic realms of the continental masses and intervening oceans involved in the Variscan colli sion. Assuming that the existing ophiolites represent the suture formed by the closure of the Rheic ocean, the units in the fo otwall to the suture correspond to the outer edge of the Good waDa continentalmargin, and the units in the hanging waD are interpreted as fragments of the conjugate margin, represented by the Me guma terrane. This correlation establishes a precise link betweencircum-Atlantic terranes, and makes it possible to draw a relatively simple sce nario of the successive tectonothermal events recorded. Following the amalgamation of Avalon to LaUl'entia, the remaining outboard terranes underwent a progressive accretion to this conti nent that ended with the collision between Laurentia and Gondwana. INTRODUCTION position in the nappe pile: basal, intermediate, granulite, amphibolite and greenschist facies. The allochthonousterranes of northwest Iberia and upper units. Because the intermediate units The ophiolitic nappes were stacked during the outcrop in five synforms or structural basins as show clear oceanicaffinities, they are referred to closure of the Rheic ocean. -
Late Devonian Peraluminous Granitic Plutons in the Canso Area, Eastern Meguma Terrane, Nova Scotia*
LATE DEVONIAN PERALUMINOUS GRANITIC PLUTONS IN THE CANSO AREA, EASTERN MEGUMA TERRANE, NOVA SCOTIA* J.D. Hill Geological Survey of Canada 601 Booth Street, Ottawa, Ontario K1A 0E8 present address: Department of Mining Engineering Technical University of Vova Scotia P.O. Box 1000, Halifax, N o v a Scotia B3J 2X4 Date Received October 10, 1987 Date Accepted January 10, 1988 Late Devonian granitic rocks are well exposed in coastal outcrops near Canso in the eastern part of the Meguma Terrane. They form numerous, relatively homogeneous plutons of peraluminous biotite-muscovite granite and minor granodiorite which are enriched in muscovite, aluminum and alkalis and depleted in biotite and cafemic components relative to comparable rocks in other Meguma Terrane granites such as the South Mountain and Musquodoboit Batholiths. Individual plutons, which crystallized between 371 and 373 Ma based on U-Pb isotopic data, show a variable relationship between composition and relative age of intrusion. This implies crystallization from two or more separate batches of slightly different magma supplied from below the current level of exposure. Highly felsic, peraluminous compositions and initial °'Sr/B°Sr ratios ranging from 0.703 to 0.709 favour magma derivation from heterogenous intermediate to felsic continental crust containing some pelitic material. Intrusions were emplaced syntectonically during the early part of a ductile shearing event related to dextral transcurrent movements in the Cobequid-Chedabucto Fault System. Des roches granitiques tardiddvoniennes forment de vastes affleurements littoraux prAs de Canso, dans la partie orientale de la LaniAre de MAguma, oil elles dAterminent des plutons nombreux, relativement homogAnes, constituAs surtout de granites pAralumineux A biotite et muscovite avec.quelque granodiorite, qui sont enrichis en muscovite, aluminium, alcalis et ddficitaires en biotite et composants cafAraiques par rapport aux roches semblables des autres granites de la LaniAre de MAguma tels que les batholites de South Mountain et de Musquodoboit. -
Meguma Terrane Revisited: Stratigraphy, Metamorphism, Paleontology, and Provenance Chris E
Document generated on 09/27/2021 5:58 a.m. Geoscience Canada Meguma Terrane Revisited: Stratigraphy, Metamorphism, Paleontology, and Provenance Chris E. White and Sandra M. Barr Volume 39, Number 1, 2012 URI: https://id.erudit.org/iderudit/geocan39_1fgs03 See table of contents Publisher(s) The Geological Association of Canada ISSN 0315-0941 (print) 1911-4850 (digital) Explore this journal Cite this document White, C. E. & Barr, S. M. (2012). Meguma Terrane Revisited:: Stratigraphy, Metamorphism, Paleontology, and Provenance. Geoscience Canada, 39(1), 8–12. All rights reserved © The Geological Association of Canada, 2012 This document is protected by copyright law. Use of the services of Érudit (including reproduction) is subject to its terms and conditions, which can be viewed online. https://apropos.erudit.org/en/users/policy-on-use/ This article is disseminated and preserved by Érudit. Érudit is a non-profit inter-university consortium of the Université de Montréal, Université Laval, and the Université du Québec à Montréal. Its mission is to promote and disseminate research. https://www.erudit.org/en/ 8 GAC–MAC 2012: FIELD GUIDE SUMMARY Meguma Terrane Revisited: phosed (greenschist to amphibolite stratigraphic differences. The forma- facies) during the Early to Middle tions of the Rockville Notch Group, as Stratigraphy, Devonian Neoacadian orogeny (ca. well as two distinct suites of mafic sills Metamorphism, 405–365 Ma), and intruded by numer- and dykes (White and Barr 2004), ous, late syntectonic to post-tectonic, occur only northwest of the shear Paleontology, and mainly Middle to Late Devonian, pera- zone (Figs. 1, 2). Provenance luminous, granitic plutons (Figs. 1, 2). -
Paleocontinental Setting for the Catskill Delta
Downloaded from specialpapers.gsapubs.org on June 30, 2014 Geological Society of America Special Paper 201 1985 Paleocontinental setting for the Catskill Delta Dennis V. Kent Lamont-Doherty Geological Observatory and Department of Geological Sciences Columbia University Palisades, New York 10964 ABSTRACT Paleomagnetic data confirm geological evidence for tropical paleolatitudes for dep- osition of the Catskill Delta and related Old Red deposits in Europe. A tectonic model constrained by paleomagnetic data suggests that the Catskill deposits are the product of the Acadian orogeny of the Northern Appalachians as a complex continent-continent collision between Armorica (Hercynian Europe), Laurentia (cratonic North America) and possibly Gondwana, with the Traveler terrane (central New England and New Brunswick) rotated and compressed in between. INTRODUCTION A low latitude setting for deposition of the mid- to late- approximately 20° farther south at this time. For example, meta- Devonian Catskill Delta has been inferred from geologic evi- volcanics in the Boston basin have magnetizations that were reset dence. The evidence indicates a depositional environment with during the Devonian and indicate a southern hemisphere paleo- warm to hot temperatures, geographically variable rainfall pat- latitude of about 23° (Schutts and others 1976). This difference in terns, relatively high evaporation rates, and generally easterly paleomagnetic directions led to the concept of the Acadia winds (Woodrow and others 1973). Paleomagnetic data confirm displaced-terrane (Kent and Opdyke 1978; 1979), one of three low paleolatitudes for eastern North America in the Devonian middle to late Paleozoic terranes now recognized in the Northern and place additional constraints on the paleocontinental configu- Appalachians on the basis of paleomagnetic data. -
Late Devonian Peraluminous Granitic Plutons in the Canso Area, Eastern Meguma Terrane, Nova Scotia J
Document generated on 09/26/2021 1:57 a.m. Atlantic Geology Late Devonian peraluminous granitic plutons in the Canso area, eastern Meguma Terrane, Nova Scotia J. D. Hill Volume 24, Number 1, April 1988 Article abstract Late Devonian granitic rocks are well exposed In coastal outcrops near Canso URI: https://id.erudit.org/iderudit/ageo24_1art02 In the eastern part of the Meguma Terrane. They form numerous, relatively homogeneous plutons of peralumlnous biotite-muscovite granite and minor See table of contents granodlorlte which are enriched In muscovite, aluminum and alkalis and depleted in biotite and cafemic components relative to comparable rocks in other Meguma Terrane granites such as the South Mountain and Publisher(s) Musquodoboit Batholiths. Individual plutons. which crystallized between 371 and 373 Ha based on U-Pb isotopic data, show a variable relationship between Atlantic Geoscience Society composition and relative age of intrusion. This Implies crystallization from two or more separate batches of slightly different magma supplied from below the ISSN current level of exposure. Highly felsic, peralumlnous compositions and initial Sr/ Sr ratios ranging from 0.703 to 0.7O9 favour magma derivation from 0843-5561 (print) heterogenous intermediate to felsic continental crust containing some pelitic 1718-7885 (digital) material. Intrusions were emplaced syntectonlcally during the early part of a ductile shearing event related to dextral transcurrent movements in the Explore this journal Cobequid-Chedabucto Fault System. Cite this article Hill, J. D. (1988). Late Devonian peraluminous granitic plutons in the Canso area, eastern Meguma Terrane, Nova Scotia. Atlantic Geology, 24(1), 11–19. All rights reserved © Atlantic Geology, 1988 This document is protected by copyright law. -
Late Paleozoic Motions of the Meguma Terrane,Nova Scotia: New Paleomagnetic Evidence
Geodynamics Series Plate Reconstruction From Paleozoic Paleomagnetism Vol. 12 LATE PALEOZOIC MOTIONS OF THE MEGUMA TERRANE,NOVA SCOTIA: NEW PALEOMAGNETIC EVIDENCE Dann J. Spariosu and Dennis V. Kent Lamont-Doherty Geological Observatory and Department of Geological Sciences, Columbia University, Palisades, New York 10964 J. Duncan Keppie Nova Scotia Department of Mines and Energy, 1690 Hollis Street, Halifax, Nova Scotia, Canada B3J 2Xl Abstract. Three rock units from southern Nova Introduction Scotia were sampled for a paleomagnetic study of the relationship of the Meguma terrane to the Differing paleomagnetic signatures of adjacent Atlantic-bordering continents during the Paleo geologic provinces have provided evidence for the zoic. These include the Si lure-ordovician White existence of terranes in the northern Appala Rock Formation volcanics, sandstones of the Lower chians that were exotic to cratonic North America Devonian Torbrook Format ion and red beds of the for portions of Paleozoic time, including Acadia Lower Carboniferous Cheverie Formation. Pro [Kent and Opdyke, 1978, 1979, 1980], Armorica gressive thermal and alternating field demagneti [Vander Voo, 1982], and the Traveler terrane zation of the White Rock basalts and rhyolites [Spariosu and Kent, 1981, in press, 1983]. Recen reveal a single component magnetization with a tly, plate tectonic models of the Appalachians mean direction of D • 149.10, I "' 24.3°, a 95 = have been constructed with the incorporation of 10°, for N = 13 sites. Rotation of the site mean the concept of "exotic" or "suspect" terranes in directions about the axis of the Torbrook the Paleozoic evolution of the orogen [Williams Syncline suggest a post-folding (post Middle and Hatcher, 1982; Keppie, in press, 1983]. -
Research Article Formation of Anorthositic Rocks Within the Blair River Inlier of Northern Cape Breton Island, Nova Scotia (Canada)
GeoScienceWorld Lithosphere Volume 2020, Article ID 8825465, 21 pages https://doi.org/10.2113/2020/8825465 Research Article Formation of Anorthositic Rocks within the Blair River Inlier of Northern Cape Breton Island, Nova Scotia (Canada) J. Gregory Shellnutt ,1 Jaroslav Dostal,2 J. Duncan Keppie,3 and D. Fraser Keppie4 1Department of Earth Sciences, National Taiwan Normal University, 88 Tingzhou Road Section 4, Taipei 11677, Taiwan 2Department of Geology, Saint Mary’s University, 923 Robie Street, Halifax, Nova Scotia, B3H 3C3, Canada 3Departamento de Geología Regional, Instituto de Geología, Universidad Nacional Autónoma de México, 04510 Mexico DF, Mexico 4Department of Energy, Government of Nova Scotia, Joseph Howe Building, 12th Floor, 1690 Hollis Street, Halifax, Nova Scotia, B3J 3J9, Canada Correspondence should be addressed to J. Gregory Shellnutt; [email protected] Received 4 June 2019; Accepted 2 April 2020; Published 0 June 2020 Academic Editor: Sarah M. Roeske Copyright © 2020 J. Gregory Shellnutt et al. Exclusive Licensee GeoScienceWorld. Distributed under a Creative Commons Attribution License (CC BY 4.0). Rocks from the Blair River inlier of Northern Cape Breton Island (Nova Scotia, Canada) have been correlated with either the Grenville basement of eastern Laurentia or the accreted Avalon terrane. Additional zircon U-Pb dates of spatially associated anorthositic dykes (425:1±2:2 Ma) and a metagabbro (423:8±2:5 Ma) from the Fox Back Ridge intrusion of the Blair River inlier reveal Late Silurian emplacement ages. Their contemporaneity suggests that they may be members of a larger intrusive ∗ complex. The anorthositic rocks have high Eu/Eu values (>2.5), and bulk compositions are similar to the mineral compositions of labradorite (An50-70) and andesine (An30-50). -
Geochronology and Plate Tectonic Geometry of Ordovician Magmatism and Terrane Accretion on the Laurentian Margin of New England Paul M
Boise State University ScholarWorks Geosciences Faculty Publications and Presentations Department of Geosciences 5-1-2017 Bridging the Gap Between the Foreland and Hinterland I: Geochronology and Plate Tectonic Geometry of Ordovician Magmatism and Terrane Accretion on the Laurentian Margin of New England Paul M. Karabinos Williams College Francis A. Macdonald Harvard University James L. Crowley Boise State University This document was originally published in the American Journal of Science by HighWire Press. Copyright restrictions may apply. doi: 10.2475/ 05.2017.01 [American Journal of Science, Vol. 317, May, 2017,P.515–554, DOI 10.2475/05.2017.01] American Journal of Science MAY 2017 BRIDGING THE GAP BETWEEN THE FORELAND AND HINTERLAND I: GEOCHRONOLOGY AND PLATE TECTONIC GEOMETRY OF ORDOVICIAN MAGMATISM AND TERRANE ACCRETION ON THE LAURENTIAN MARGIN OF NEW ENGLAND PAUL KARABINOS*,†, FRANCIS A. MACDONALD**, and JAMES L. CROWLEY*** ABSTRACT. U-Pb dates on magmatic and detrital zircon from samples in the hinterland of the Taconic orogen place new constraints on the timing and plate tectonic geometry of terrane accretion and magmatic arc activity. The Moretown terrane, a Gondwanan-derived exotic block, extends from the Rowe Schist-Moretown Formation contact in the west to the Bronson Hill arc in the east. Arc-related plutonic and volcanic rocks formed above an east-dipping subduction zone under the western leading edge of the Moretown terrane from approximately 500 to 475 Ma, until collision with hyperextended distal fragments of Laurentia, represented by the Rowe Schist, at 475 Ma. Magmatic arc rocks formed during this interval are primarily located in the Shelburne Falls arc, although some are also located in the Bronson Hill arc to the east. -
Late Paleozoic Strike-Slip Faults in Maritime Canada and Their Role In
1 Late Paleozoic strike-slip faults in Maritime Canada and their role 2 in the reconfiguration of the northern Appalachian orogen 3 John W.F. Waldron1, Department of Earth and Atmospheric Sciences, University of Alberta, 4 Edmonton AB Canada 5 Sandra M. Barr, Department of Earth and Environmental Science, Acadia University, Wolfville 6 NS, Canada 7 Adrian F. Park2, Department of Earth Sciences, University of New Brunswick, Fredericton, NB, 8 Canada 9 Chris E. White, Nova Scotia Department of Natural Resources, Halifax, NS, Canada 10 James Hibbard, Department of Marine, Earth and Atmospheric Sciences, North Carolina State 11 University, Raleigh, NC, USA 12 13 1Corresponding author: John W.F. Waldron, Department of Earth and Atmospheric Sciences, 14 ESB 1-26, University of Alberta, Edmonton, AB T6G2E3 Canada ([email protected]) 15 2Current address 116 Jaffrey Street, Fredericton, NB, Canada 16 17 1 18 Key points 19 Late Paleozoic dextral faults dissect northern Appalachians 20 NE-SW and E-W dextral faults active at different times 21 Restoration of strike slip necessary for understanding orogen 22 Abstract 23 Major late Paleozoic faults, many with documented strike-slip motion, have dissected the 24 Ordovician - Devonian Appalachian orogen in the Maritime Provinces of Atlantic Canada. 25 Activity alternated between east-west faults (Minas trend) and NE-SW faults (Appalachian 26 trend). NW-SE faults (Canso trend) were probably conjugate to Minas-trend faults. Major dextral 27 movement, on faults with Appalachian trend, in total between 200 and 300 km, began in the Late 28 Devonian. This movement initiated the Maritimes Basin in a transtensional environment at a 29 releasing bend formed around a promontory in the Laurentian margin, and thinned the crust, 30 accounting for the major subsidence of the basin.