Studies on the Oxygen Toxicity of Probiotic Bacteria with Reference to Lactobacillus Acidophilus and Bifidobacterium Spp
Total Page:16
File Type:pdf, Size:1020Kb
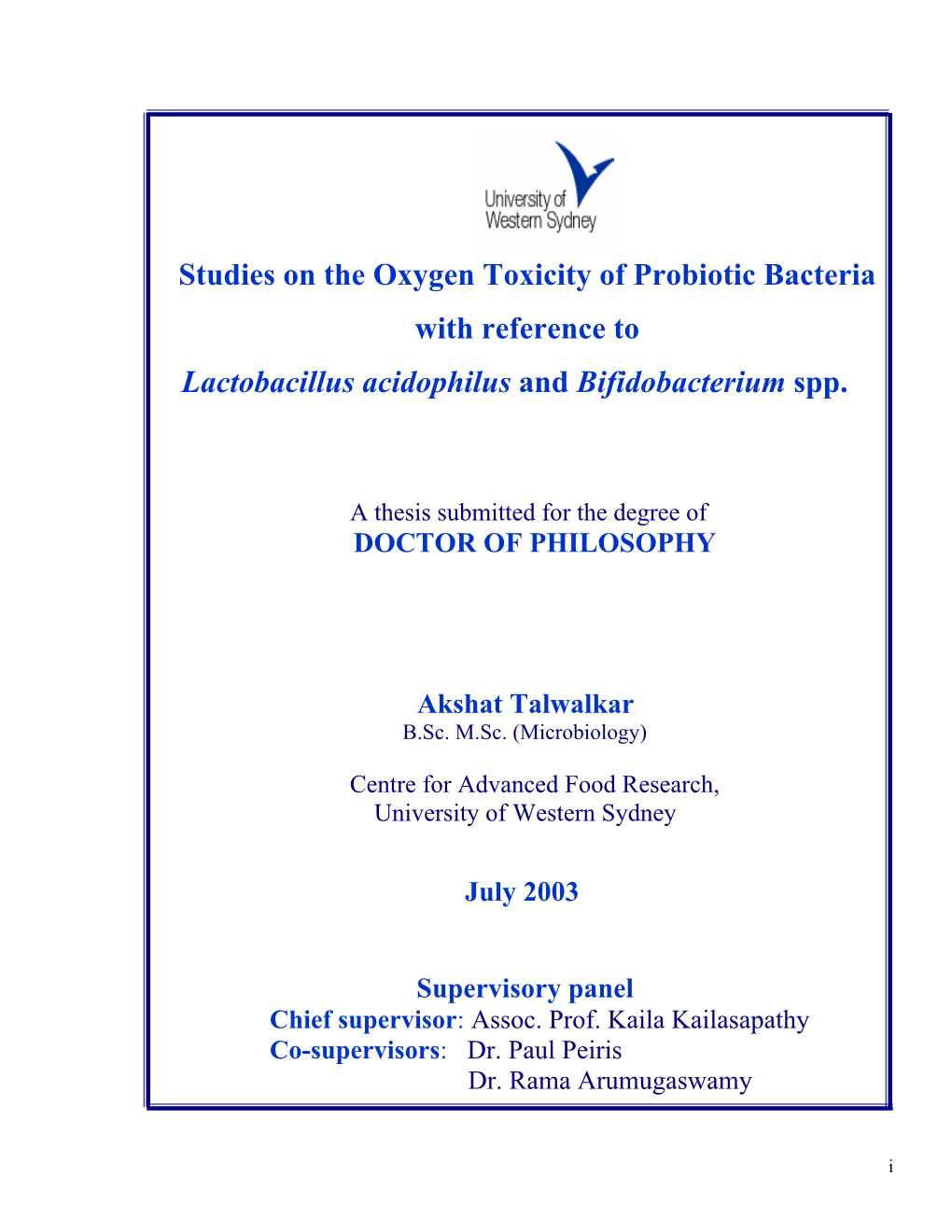
Load more
Recommended publications
-
Project Agape September 2020 #2 Newsletter
Subscribe Past Issues Translate RSS View this email in your browser Agricultural Development on the Rise September Newsletter #2 Dear <<First Name>>, The months of August and September recorded an increase in productivity regarding the “Agricultural Development Project,” along with the “Distribution Project.” Four socially vulnerable families in four diverse villages received four cows that were delivered to them. One of the cows was named Deborah after the request of a person who donated it to a family connected with the Project AGAPE. Hrair Tatincyan and his family received Deborah in a village called Karegah, located about ten miles from Berdzor. The former is a veteran, having several wounds from the 4-day war of 2016. Hrair did not stay indifferent to the danger knocking at the eastern and southwestern gates of his homeland, so without any hesitation, he decided to join one of the many groups of volunteers to protect his country’s remnants. His wife is an account, who unfortunately cannot find a job in the village. The family struggles to live on the pension that Hrair receives as a handicapped person. “I cannot sit without doing anything; I should work. I am so grateful for such an amazing contribution to my family. The only way to show my gratitude is to take good care of the cow that will feed my children, grow my farm, and then help a family like ours...” – Hrair Another family that received a cow belongs to Andranik Mikaelyan, living in Vakunis, a village located in the northeastern part of Kashatagh. Andranik and his wife Alina have eight children: five sons and three daughters. -
The Evolution, Processing, Varieties and Health Benefits of Yogurt
International Journal of Scientific and Research Publications, Volume 4, Issue 4, April 2014 1 ISSN 2250-3153 The evolution, processing, varieties and health benefits of yogurt W.A.D.V. Weerathilake*, D.M.D. Rasika*, J.K.U. Ruwanmali* and M.A.D.D. Munasinghe** * Department of Livestock & Avian Sciences, Faculty of Livestock Fisheries & Nutrition, Wayamba University of Sri Lanka, Makandura, Gonawila 60170, Sri Lanka **Faculty of Agriculture, University of Peradeniya, Peradeniya 20400, Sri Lanka Abstract- Yogurt or yoghurt is one of the most popular fermented producing bacteria, Lactobacillus bulgaricus and Streptococcus dairy products worldwide which has great consumer thermophilus [3].Yogurt should contain at least 3.25% of milk fat acceptability due to its health benefits other than its basic and 8.25% of Milk Solids Non Fat (MSNF) with a titratable nutrition. In general, yogurt is considered as a nutrition-dense acidity of not less than 0.9 percent, expressed as lactic acid [3]. food due to its nutrient profile and is a rich source of calcium that The composition requirement for milk fat and MSNF is applied provides significant amounts of calcium in bio-available form. In to the yogurt prior to the addition of bulky flavoring ingredients addition, it provides milk proteins with a higher biological value according to the USDA specifications for yogurt [4]. and provides almost all the essential amino acids necessary to Traditionally yogurt is made of cow, water buffalo, goat and maintain good health.Yogurt is considered as a probiotic carrier sheep milk. However, milk from mare and camel is also used in food that can deliver significant amounts of probiotic bacteria yogurt making in some of the regions in the world. -
5Iffirl OUTLINE
' NATI-1-D i\1 ITIV LTD V1:10 - 0 0 0 0 0 0 0 5iffirl OUTLINE. Milk: 1. Introduction. 2. Sourceo 3. Composition. 4. Nutritive and Dietetic Value. 5. Digestfon. 6. Products and By-products. a. Cream b. Butter c. Cheese d. Skim -milk e. Butter -milk 7. Properties in Relation to a. Heat b. Cold c. Bacteria 8. Adulteration and Preservatives. 9. Modifications: a. Condensed Yilk b. Koumiss 10. Care of Milk: a. Aeration b. Pasteurization c. Sterilization MILK. The use of milk is general and not limited to any class or locality; it is regarded as a necessity by almost every family and because of this, we cannot glean too much information regard- ing it. This is doubly important when we think of the failure of Americans to appreciate the food value of milk and its products. Although this is one of the greatest dairy countries in the world, it does not lead in the per capita consumption of dairy products. In some of the older European countries two or three times as much milk and cheese is consumed per capita as in the United States. However, the average consumption of milk is high in many parts of this country, and, assisted by improved methods of production and transportation, it seems to be increasing. Milk contains all the ingredients needed for nourishment; that is, it furnishes the materials which build up the body and keep it in repair, and also those which supply it with fuel to keep it warm. and to furnish the energy needed to do its work. -
Starter Cultures Aibi® for Fermented Milk Products Manufacturing
Starter cultures AiBi® for fermented milk products manufacturing SOYUZSNAB-IS A LEADEROF INNOVATIONS INBACTERIAL CULTURES’ PRODUCTION SOYUZSNAB Group is a world leading innovative company, that has made a progressive leap in the development of bacterial cultures production and development. SOYUZSNAB holds a strong position in the Russian and International marketplace of starter and other bacterial cultures by its competitive advantage. These advantages include unique collection of microbial strains which are permanently being replenished; «Know-how» in production cycle; emphasis of final product’s sensory characteristics; unconditional refusal of using genetically modified organisms; perfect warranty system. SCIENTIFIC BASIS The production of bacterial cultures at SOYUZSNAB Group is based on long-term microbiological research. Continuous work by SOYUZSNAB Group Research and Development Complex team has resulted in the development of acollection of 3000 censoring microbial strains.The natural sources of microorganisms are from different places from all over the world. A rich diversity of initial strains allowed to make for a unique combination of starter cultures which takes into account of the specificity of a concrete region, the requirements specification and the taste preferences of the consumers. «KNOW-HOW» IN PRODUCTION The fermenter lines, where biomass of microorganisms grow, are completely automated and factors out humans in the technical process. Science and Production Association «Zelenyi Linii» started using its own creation in concentration of biomass using a shielding medium for microorganisms and sublimation process. Qualified experts of SOYUZSNAB Group create unique, direct analogs in the world’s market for multi-component starter cultures. The composition of starter culture contains up to 15 types of microorganisms and many types of strains. -
Biochemistry of Milk
ФГБОУ ВО НОВОСИБИРСКИЙ ГАУ БИОЛОГО-ТЕХНИЧЕСКИЙ ФАКУЛЬТЕТ Biochemistry of milk Методические указания по выполнению самостоятельной и контрольных работ Новосибирск 2017 УДК 637.12.047(075) ББК 36.95:28.072 C687 Кафедра ветеринарной генетики и биотехнологии Составитель: Короткевич О.С., д.б.н., профессор Рецензент: Бокова Т.И., д.б.н., профессор, зав. кафедры химии НГАУ Biochemistry of milk: методические указания по выполнению самостоятельной и контрольных работ/ сост. Короткевич О.С.; Новосиб. гос. аграр. ун-т. Биолого-технологический факультет.- Новосибирск, 2017. - 47 с. Методические указания предназначены для бакалавров, магистров и аспирантов очной и заочной форм обучения Биолого-технологического факультета, обучающихся по направлению подготовки 19.03.04. Технология продукции и организация общественного питания, Изложены основные разделы курса «Biochemistry of milk» с указанием видов и форм контроля тем, выносимых для самостоятельной работы бакалавров, магистров и аспирантов очной и заочной форм обучения и выполнения контрольных. Приведены словарь-терминов, библиографический список, вопросы для контроля. Методические указания утверждены и рекомендованы к изданию на заседании учебно-методического совета БТФ (протокол № 2 от 1 марта 2017 года) © Новосибирский государственный аграрный университет, 2017 Содержание 1. Введение.....................................................................................................5 1.1. Цели и задачи учебной дисциплины....................................................5 2. Содержание отдельных -
WO 2018/142193 Al 09 August 2018 (09.08.2018) W !P O PCT
(12) INTERNATIONAL APPLICATION PUBLISHED UNDER THE PATENT COOPERATION TREATY (PCT) (19) World Intellectual Property Organization International Bureau (10) International Publication Number (43) International Publication Date WO 2018/142193 Al 09 August 2018 (09.08.2018) W !P O PCT (51) International Patent Classification: (72) Inventor: FODELIANAKIS, Stylianos; c/o King Abdul A23C 9/12 (2006.01) A23C 9/127 (2006.01) lah University of Science and Technology, Technology A23C 9/123 (2006.01) Transfer Office, 4700 King Abdullah University of Science and Technology, Thuwal, 23955-6900 (SA). (21) International Application Number: PCT/IB20 17/053095 (81) Designated States (unless otherwise indicated, for every kind of national protection available): AE, AG, AL, AM, (22) International Filing Date: AO, AT, AU, AZ, BA, BB, BG, BH, BN, BR, BW, BY, BZ, 25 May 2017 (25.05.2017) CA, CH, CL, CN, CO, CR, CU, CZ, DE, DJ, DK, DM, DO, (25) Filing Language: English DZ, EC, EE, EG, ES, FI, GB, GD, GE, GH, GM, GT, HN, HR, HU, ID, IL, IN, IR, IS, JP, KE, KG, KH, KN, KP, KR, (26) Publication Language: English KW, KZ, LA, LC, LK, LR, LS, LU, LY, MA, MD, ME, MG, (30) Priority Data: MK, MN, MW, MX, MY, MZ, NA, NG, NI, NO, NZ, OM, 62/455,079 06 February 2017 (06.02.2017) US PA, PE, PG, PH, PL, PT, QA, RO, RS, RU, RW, SA, SC, SD, SE, SG, SK, SL, SM, ST, SV, SY,TH, TJ, TM, TN, TR, (71) Applicant: KING ABDULLAH UNIVERSITY OF TT, TZ, UA, UG, US, UZ, VC, VN, ZA, ZM, ZW. -
Eleek Eeae Peg Aada) MASTER of HOME ECONOMICS
Ele ek ee ae Peg aad a) MASTER OF HOME ECONOMICS BY LYDIA ZAE NORTHRUP i 1913 @ ; c 3 Lge 0 hae, KB AN ORE ORS isk Beas AM Ke Fo sry! a yn GS Oe on KOM ae, a = 5 “¢ ms SAP TND A ae NS? en OC aae YAR na Pies Hs TOY OO RNERG ate . >) > . RAN ey ry . ‘, ee ae, . see ot SAK a ah ay pt Abe net ed S86 935 nN 12 e 1) ee, 7 Ore 2) ‘5 Sort en ao Wert ap, ‘ i & Bh 1 Ree etd Raat OF £2 eS fs see RoR a aN BT ; 2 Oy: € wire * petto yen Vit LR uit . Pye ; Hic wi" ‘ ; 7,32 eaten IR USS 4 ey EN PORE SSN eh oe 2 ae Rishon sie FON COI Tel ROMA I Ae AGM OSE I eR AO ok ane ee SG %f ei Se Nimes 3s Se eh Re en ¢ rd ose oO ; ig 8 vs sn THO 4% c 3 ~ e AY I Ae ; Ke SCN Z ig ay ay Shan: ie ap ee, Ki br ars oe We aLeeae AYER @ Reh Yi YE i! OCR : CR Re toes : ar 2 5? ep . “ es pA role we das DAG ® A a ts s de THKH UTILITY OF LACTIC BACTERIA. Thesis for the derree of MASTER OF HOME EBFCOWOMICS . by Lydia zoe Northrup =a on ww we 4 oe OR 1913 THESIS THE UTILITY OF LACTIC BACTERIA. Pernaps no other single group of micro6r- ganisms has such a wide distribution in nature and in addition, such a range of usefulness sas has the group of lactic acid forming bacteria which sare re~ sponsible for the cheracteristic fermentative change in milk known as curdling. -
Sensory Characteristics and Classification of Commercial and Experimental Plain Yogurts
SENSORY CHARACTERISTICS AND CLASSIFICATION OF COMMERCIAL AND EXPERIMENTAL PLAIN YOGURTS by MARISSA BROWN B.S., University of Delaware, 2008 A THESIS submitted in partial fulfillment of the requirements for the degree MASTER OF SCIENCE Food Science KANSAS STATE UNIVERSITY Manhattan, Kansas 2010 Approved by: Major Professor Dr. Delores Chambers Department of Human Nutrition Abstract This research aimed to determine the sensory characteristics of commercially-available plain yogurts and examine how three "more sustainable" prototypes compared. Three experimental non-fat set-style yogurts were provided – one control and two samples that differed in fermentation time. These shortened fermentation times could result in energy reductions and potentially substantiate a “sustainable” marketing claim, a concept gaining traction with consumers. Twenty-six commercially-available yogurts varying in percent milk fat, milk type (organic or conventional), and processing (set- style, stirred, or strained/Greek-style) were also included. Using descriptive sensory analysis, a six-person highly-trained panel scored the intensity of 25 flavor, six texture, four mouthfeel, and two mouthcoating attributes on a 15-point numerical scale. Three replications were conducted, and all samples were tested at least 10 days prior to the end of their shelf-lives. The samples differed for 19 flavor and all texture, mouthfeel, and mouthcoating attributes. Cluster analysis indicated approximately seven flavor and five texture (texture, mouthfeel, and mouthcoating combined) clusters, resulting in 15 unique combinations of flavor and texture. Although no legal definitions exist for “sustainable,” the prototypes’ sensory characteristics were comparable to those of top- selling yogurts indicating potential market viability. This research also demonstrated potential growth opportunities. -
Copyrighted Material
1 History and consumption trends Ramesh C. Chandan Global Technologies, Inc. , Coon Rapids , Minnesota, USA 1.1 Overview of the world dairy industry According to the UN Food and Agriculture Organization (FAO, 2011), the world production of milk in 2009 was 701.4 million metric tons (MT). This was estimated to increase to 713.6 million MT in 2010 and to 727.6 million MT in 2011. India is the largest producer of milk (including milk of cows and water buffaloes) in the world, with an estimated 121.7 million MT in the year 2011. The 2009 world production of cow milk in the selected countries shown in Table 1.1 was 432.7 million MT. The documented number of cows was 129 296 thousand heads. Individual cow-milk yield varies widely around the world. In 2009, the USA and Japan were the most efficient milk producers, with 9.33 MT/cow, followed by Canada, with a yield of 8.46 MT per cow. Milk yield was lowest in India (1.13 MT/head), followed by Brazil (1.67 MT/head) and Mexico (1.70 MT/head). 1.2 Milk production in the USA The trend in the last decade indicates a noticeable decrease in dairy-cow population, from 9.151 million heads in the year 1998 to 9.117 million heads in 2010 (Table 1.2 ). In the year 2010, 9.117 million cows produced 87.46 million MT (192 819 million pounds) of milk (IDFA, 2011). Table 1.2 also shows that during the period 1998–2010 there is a steady increase in milkCOPYRIGHTED production per cow, from 7.79 MT MATERIAL (17 185 pounds) to 9.59 MT (21 149 pounds). -
Preparation of Frozen Fermented Foods Using
_ <v Europaisches 111111111111111111111111111111 JGV/l Eur°Pean Patent Office <*S Office europeen des brevets (11) EP 0 785 727 B1 (12) EUROPEAN PATENT SPECIFICATION (45) Date of publication and mention (51) int. CI.6: A23L 1/03, A23C 9/123, of the grant of the patent: A23C 19/032 12.05.1999 Bulletin 1999/19 (86) International application number: ..... - ...number: 95933279.2 (21) Application PCT/CA95/00568 (22) Date of filing: 11.10.1995 x 7 3 (87)/Q-,X International. 4 4..... publication 4. number: WO 96/11586 (25.04.1996 Gazette 1996/18) (54) PREPARATION OF FROZEN FERMENTED FOODS USING ANTIFREEZE POLYPEPTIDE- EXPRESSING MICROORGANISMS HERSTELLUNG VON GEFRORENEN FERMENTIERTEN NAHRUNGSMITTELN MIT GEFRIERSCHUTZ-POLYPEPTIDE EXPREMIERENDEN MIKROORGANISMEN PREPARATION D' ALIMENTS FERMENTES GLACES EN UTILISANT DES MICRO-ORGANISMES EXPRIMANT UN POLYPEPTIDE ANTIGEL (84) Designated Contracting States: • HEW, Choy, L. AT BE CH DE DK ES FR GB GR IE IT LI LU MC NL Thornhill, Ontario L4J 2A3 (CA) PTSE • JOSHI, Shashikant, B. Toronto, Ontario M5G 1 H4 (CA) (30) Priority: 12.10.1994 US 321991 • WU, Yaling St. John's, Newfoundland A1 A 2V2 (CA) (43) Date of publication of application: 30.07.1997 Bulletin 1997/31 (74) Representative: Thomson, Paul Anthony et al (73) Proprietors: Potts, Kerr & Co. • HSC Research and Development Limited 15, Hamilton Square Partnership Birkenhead Merseyside L41 6BR (GB) Toronto, Ontario M5G 1X8 (CA) • SEABRIGHT CORPORATION LIMITED (56) References cited: St. John's, Newfoundland, A1C 5S7 (CA) EP-A- 0 424 771 EP-A- 0 443 543 WO-A-90/13571 WO-A-94/03617 (72) Inventors: • FLETCHER, Garth, L. -
Fermente Süt Ürünleri Teknolojisi I
03.01.2017 ÇEŞİTLİ FERMENTE SÜT YOĞURT ÜRÜNLERİ HAZIRLAYAN: ÖĞR.GÖR.ŞEVKİ ÇETİNER SÜZME YOĞURT (strained yogurt) AYRAN 1 03.01.2017 KEFİR – ASYA ve Kuzeydoğu Avrupa ÇÖKELEK KIMIZ – ORTA ASYA SHUBAT – ORTA ASYA QATYG – ORTA ASYA 2 03.01.2017 QUARK – Almanya ve Kuzey Avrupa ASİDOFİLUSLU SÜT – KUZEY AMERİKA ve AVRUPA Kærnemælk – DANİMARKA LEBBEN – ARABİSTAN ve MISIR KİSHK – ARABİSTAN Ymer – DANİMARKA 3 03.01.2017 Boruga – DOMİNİK CUMHURİYETİ Piimä – FİNLANDİYA Viili – FİNLANDİYA kiselo mlyako – BULGARİSTAN Matsoni – GÜRCİSTAN Xynogala – YUNANİSTAN Aludttej – MACARİSTAN Sauermilch – ALMANYA 4 03.01.2017 Jocoque – MEKSİKA YAKULT – JAPONYA leche agria – Nicaragua CALPIS – JAPONYA Surmelk (kulturmelk) – NORVEÇ Tjukkmjølk– NORVEÇ Karnemelk (buttermilk) – HOLLANDA 5 03.01.2017 Lassi – PAKİSTAN Acidofilne – POLANYA Twaróg – POLANYA Dahi – PAKİSTAN Lapte acru – ROMANYA Prostokvasha– RUSYA, UKRAYNA, BELARUS Ryazhenka– RUSYA, UKRAYNA, BELARUS Lapte bătut – ROMANYA 6 03.01.2017 Varenets – RUSYA, UKRAYNA, BELARUS Blaand – İSKOÇYA Kivuguto – RUANDA Tvorog – RUSYA, UKRAYNA, BELARUS A-fil – İSVEÇ Amasi – GÜNEY AFRİKA kiselo mleko – SIRBİSTAN Filmjölk – İSVEÇ 7 03.01.2017 långfil – İSVEÇ Clabber – ABD Lacto – Zimbabwe Kiselo, mlijeko ve kefir – Bosna Hersek Urubu – Brundi Turkic countries (ayran) –Türkiye 8 03.01.2017 Kule, naoto, maziwa ve lala– Kenya Rob – Sudan Ergo – Etyopya Kaymak – Central Asia Smetana – Central & Eastern Europe kermaviili – Finlandiya mileram/kiselo vrhnje – Hırvatistan crème fraîche – Fransa kiselo 9 03.01.2017 sýrður rjómi – İzlanda -
МEНЮ Menu ЗАВТРАКИ I BREAKFAST
МEНЮ Menu ЗАВТРАКИ I BREAKFAST ОМЛЕТ С ЛОСОСЕМ 390 q ЯЙЦО БЕНЕДИКТ 490 q ЯЙЦО БЕНЕДИКТ 360 q С ЛОСОСЕМ С БЕКОНОМ SALMON OMELETTE ЗАВТРАКИ EGGS BENEDICT WITH SALMON EGGS BENEDICT WITH HAM Breakfast ПОНЧИКИ 380 q С ЗАВАРНЫМ КРЕМОМ Подаются с лимонадом из тархуна CUSTARD DONUTS Served with tarragon lemonade ОМЛЕТ 260 q С ОВОЩАМИ И СЫРОМ CHEESE AND VEGETABLE OMELETTE СКРЕМБЛ 250 q SCRAMBLED EGGS ЯИЧНИЦА- 150 q ГЛАЗУНЬЯ SUNNY SIDE UP EGGS ЗАВТРАКИ I BREAKFAST ЗАВТРАКИ I BREAKFAST q q РИСОВАЯ КАША 290 СЭНДВИЧ 490/390 ОЛАДЬИ 390 q С КОКОСОВЫМ МОЛОКОМ, на выбор с лососем или куриным рулетом ИЗ КАБАЧКОВ С ЛОСОСЕМ МАНГОВЫМ СОУСОМ И ГРЕЙПФРУТОМ SANDWICH MARROW FRITTERS WITH SALMON with salmon RICE PORRIDGE WITH COCONUT or chicken roll MILK, MANGO SAUCE to your choice AND GRAPEFRUIT ПШЕННАЯ КАША 190 q С ТЫКВОЙ И ГРЕЦКИМ ОРЕХОМ MILLET PORRIDGE q WITH PUMPKIN AND WALNUTS ГРЕЧНЕВАЯ КАША 190 q СО СЛИВОЧНЫМ МАСЛОМ КРУАССАН 290/350 на выбор с ветчиной ДОМАШНИЙ 290 q BUCKWHEAT PORRIDGE и сыром или с лососем WITH BUTTER ТВОРОГ С МАЛИНОЙ CROISSANT HOMEMADE COTTAGE CHEESE with ham and cheese WITH RASPBERRY or with salmon to your choice ОВСЯНАЯ КАША 210 q С КАРАМЕЛИЗИРОВАННЫМ ЯБЛОКОМ OATMEAL PORRIDGE НАПИТКИ WITH CARAMELIZED APPLE КОФЕ ПО-ТБИЛИССКИ 170 q АЙРАНИ 190 q Кофе, сваренный в турке на песке Напиток на основе мацони ОТ ШЕФ-ПОВАРА по старинному рецепту со свежей зеленью и огурцом Drinks from the Chef TBILISI-STYLE COFFEE AYRAN Coffee made in Turkish pot Matzoon-based drink on sand by an old recipe with fresh herbs and cucumbers q ЖАРЕНАЯ КОЛБАСА 310 БЛИНЫ