An Sirna-Based Functional Genomics Screen for the Identification of Regulators of Ciliogenesis and Ciliopathy Genes
Total Page:16
File Type:pdf, Size:1020Kb
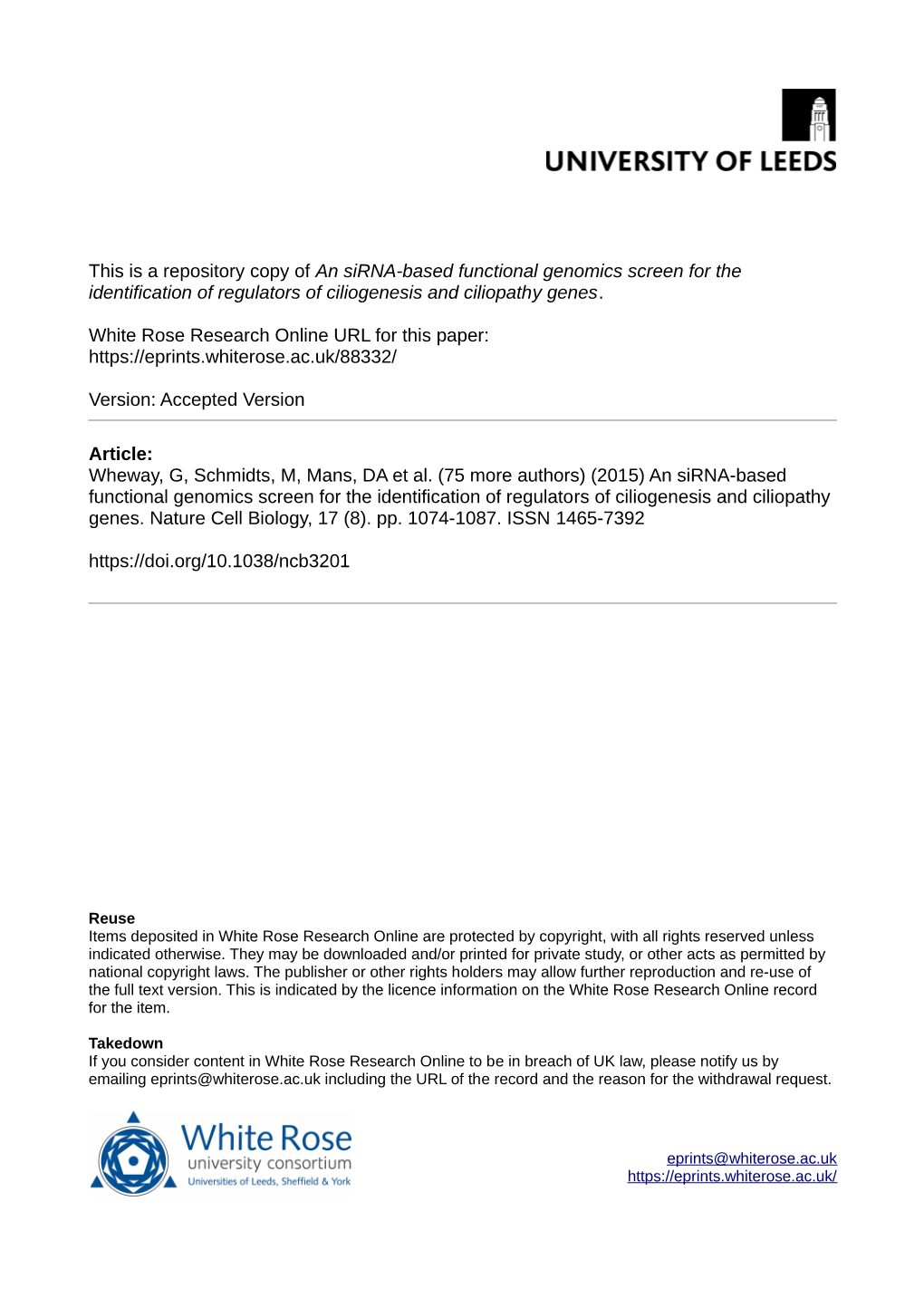
Load more
Recommended publications
-
The Vertebrate Retina Contains Two Types of Photoreceptors: Rods That
JOURNAL OF NEUROCHEMISTRY | 2009 | 108 | 91–101 doi: 10.1111/j.1471-4159.2008.05739.x *Department of Anatomy, School of Medicine, Tokyo Women’s Medical University, Tokyo, Japan Genetics and Development Division, Toronto Western Research Institute, University Health Network, Department of Ophthalmology and Visual Sciences and Department of Laboratory Medicine and Pathobiology, University of Toronto, Toronto, Ontario, Canada àUniversity of Ottawa Eye Institute and Ottawa Health Research Institute, Ottawa, Ontario, Canada Abstract The retinas of staggerer mice, carrying a null mutation of Color vision is supported by retinal cone photoreceptors that, RORa, show significant down-regulation of Opn1sw, in most mammals, express two photopigments sensitive to Opn1mw, and Arr3. RORa acts in synergy with cone-rod short (S-opsin) or middle (M-opsin) wavelengths. Expression homeobox transcription factor (Crx), to activate the Opn1sw of the Opn1sw and Opn1mw genes, encoding S-opsin and promoter in vitro. Chromatin immunoprecipitation assays re- M-opsin, respectively, is under the control of nuclear veal that RORa directly binds to the Opn1sw promoter, receptors, including thyroid hormone receptor b2 (TRb2), Opn1mw locus control region, and the Arr3 promoter in vivo. retinoid X receptor c (RXRc), and RORb, a member of the Our data suggest that RORa plays a crucial role in cone retinoic acid receptor-related orphan receptor (ROR) family. development by directly regulating multiple cone genes. We now demonstrate that RORa, another member of the ROR Keywords: arrestin, cone photoreceptor, opsin, retina, family, regulates Opn1sw, Opn1mw, as well as Arr3 (cone RORa, staggerer. arrestin) in the mouse retina. RORa expression is detected in J. -
Title a New Centrosomal Protein Regulates Neurogenesis By
Title A new centrosomal protein regulates neurogenesis by microtubule organization Authors: Germán Camargo Ortega1-3†, Sven Falk1,2†, Pia A. Johansson1,2†, Elise Peyre4, Sanjeeb Kumar Sahu5, Loïc Broic4, Camino De Juan Romero6, Kalina Draganova1,2, Stanislav Vinopal7, Kaviya Chinnappa1‡, Anna Gavranovic1, Tugay Karakaya1, Juliane Merl-Pham8, Arie Geerlof9, Regina Feederle10,11, Wei Shao12,13, Song-Hai Shi12,13, Stefanie M. Hauck8, Frank Bradke7, Victor Borrell6, Vijay K. Tiwari§, Wieland B. Huttner14, Michaela Wilsch- Bräuninger14, Laurent Nguyen4 and Magdalena Götz1,2,11* Affiliations: 1. Institute of Stem Cell Research, Helmholtz Center Munich, German Research Center for Environmental Health, Munich, Germany. 2. Physiological Genomics, Biomedical Center, Ludwig-Maximilian University Munich, Germany. 3. Graduate School of Systemic Neurosciences, Biocenter, Ludwig-Maximilian University Munich, Germany. 4. GIGA-Neurosciences, Molecular regulation of neurogenesis, University of Liège, Belgium 5. Institute of Molecular Biology (IMB), Mainz, Germany. 6. Instituto de Neurociencias, Consejo Superior de Investigaciones Científicas and Universidad Miguel Hernández, Sant Joan d’Alacant, Spain. 7. Laboratory for Axon Growth and Regeneration, German Center for Neurodegenerative Diseases (DZNE), Bonn, Germany. 8. Research Unit Protein Science, Helmholtz Centre Munich, German Research Center for Environmental Health, Munich, Germany. 9. Protein Expression and Purification Facility, Institute of Structural Biology, Helmholtz Center Munich, German Research Center for Environmental Health, Munich, Germany. 10. Institute for Diabetes and Obesity, Monoclonal Antibody Core Facility, Helmholtz Center Munich, German Research Center for Environmental Health, Munich, Germany. 11. SYNERGY, Excellence Cluster of Systems Neurology, Biomedical Center, Ludwig- Maximilian University Munich, Germany. 12. Developmental Biology Program, Sloan Kettering Institute, Memorial Sloan Kettering Cancer Center, New York, USA 13. -
The Ciliated Cell Transcriptome A
THE CILIATED CELL TRANSCRIPTOME A DISSERTATION SUBMITTED TO THE DEPARTMENT OF BIOLOGICAL SCIENCES AND THE COMMITTEE ON GRADUATE STUDIES OF STANFORD UNIVERSITY IN PARTIAL FULFILLMENT OF THE REQUIREMENTS FOR THE DEGREE OF DOCTOR OF PHILOSOPHY Ramona Hoh March 2010 ! © 2010 by Ramona Amy Hoh. All Rights Reserved. Re-distributed by Stanford University under license with the author. This work is licensed under a Creative Commons Attribution- Noncommercial 3.0 United States License. http://creativecommons.org/licenses/by-nc/3.0/us/ This dissertation is online at: http://purl.stanford.edu/sk794dv5857 ii I certify that I have read this dissertation and that, in my opinion, it is fully adequate in scope and quality as a dissertation for the degree of Doctor of Philosophy. Timothy Stearns, Primary Adviser I certify that I have read this dissertation and that, in my opinion, it is fully adequate in scope and quality as a dissertation for the degree of Doctor of Philosophy. Mark Krasnow I certify that I have read this dissertation and that, in my opinion, it is fully adequate in scope and quality as a dissertation for the degree of Doctor of Philosophy. Maxence Nachury I certify that I have read this dissertation and that, in my opinion, it is fully adequate in scope and quality as a dissertation for the degree of Doctor of Philosophy. William Nelson Approved for the Stanford University Committee on Graduate Studies. Patricia J. Gumport, Vice Provost Graduate Education This signature page was generated electronically upon submission of this dissertation in electronic format. An original signed hard copy of the signature page is on file in University Archives. -
Mechanisms of Acetylcholine Receptor Loss in Myasthenia Gravis
J Neurol Neurosurg Psychiatry: first published as 10.1136/jnnp.43.7.601 on 1 July 1980. Downloaded from Journal of Neurology, Neurosurgery, and Psychiatry, 1980, 43, 601-610 Mechanisms of acetylcholine receptor loss in myasthenia gravis DANIEL B DRACHMAN, ROBERT N ADAMS, ELIS F STANLEY, AND ALAN PESTRONK From the Department of Neurology, Johns Hopkins University School of Medicine, Baltimore, Maryland, USA SUMMARY The fundamental abnormality affecting the neuromuscular junctions of myasthenic patients is a reduction of available AChRs, due to an autoimmune attack directed against the receptors. Antibodies to AChR are present in most patients, and there is evidence that they have a predominant pathogenic role in the disease, aided by complement. The mechanism of antibody action involves acceleration of the rate of degradation of AChRs, attributable to cross-linking of the receptors. In addition, antibodies may block AChRs, and may participate in producing destructive changes, perhaps in conjunction with complement. The possibility that cell-mediated mechanisms may play a role in the autoimmune responses of some myasthenic patients remains to be explored. Although the target of the autoimmune attack in myasthenic patients is probably always the acetyl- Protected by copyright. choline receptors, it is not yet clear which of these immune mechanisms are most important. It is likely that the relative role of each mechanism varies from patient to patient. One of the goals of future research will be to identify the relative importance of each -
Synergistic Genetic Interactions Between Pkhd1 and Pkd1 Result in an ARPKD-Like Phenotype in Murine Models
BASIC RESEARCH www.jasn.org Synergistic Genetic Interactions between Pkhd1 and Pkd1 Result in an ARPKD-Like Phenotype in Murine Models Rory J. Olson,1 Katharina Hopp ,2 Harrison Wells,3 Jessica M. Smith,3 Jessica Furtado,1,4 Megan M. Constans,3 Diana L. Escobar,3 Aron M. Geurts,5 Vicente E. Torres,3 and Peter C. Harris 1,3 Due to the number of contributing authors, the affiliations are listed at the end of this article. ABSTRACT Background Autosomal recessive polycystic kidney disease (ARPKD) and autosomal dominant polycystic kidney disease (ADPKD) are genetically distinct, with ADPKD usually caused by the genes PKD1 or PKD2 (encoding polycystin-1 and polycystin-2, respectively) and ARPKD caused by PKHD1 (encoding fibrocys- tin/polyductin [FPC]). Primary cilia have been considered central to PKD pathogenesis due to protein localization and common cystic phenotypes in syndromic ciliopathies, but their relevance is questioned in the simple PKDs. ARPKD’s mild phenotype in murine models versus in humans has hampered investi- gating its pathogenesis. Methods To study the interaction between Pkhd1 and Pkd1, including dosage effects on the phenotype, we generated digenic mouse and rat models and characterized and compared digenic, monogenic, and wild-type phenotypes. Results The genetic interaction was synergistic in both species, with digenic animals exhibiting pheno- types of rapidly progressive PKD and early lethality resembling classic ARPKD. Genetic interaction be- tween Pkhd1 and Pkd1 depended on dosage in the digenic murine models, with no significant enhancement of the monogenic phenotype until a threshold of reduced expression at the second locus was breached. -
Investigating Cone Photoreceptor Development Using Patient-Derived NRL Null Retinal Organoids
ARTICLE https://doi.org/10.1038/s42003-020-0808-5 OPEN Investigating cone photoreceptor development using patient-derived NRL null retinal organoids Alyssa Kallman1,11, Elizabeth E. Capowski 2,11, Jie Wang 3, Aniruddha M. Kaushik4, Alex D. Jansen2, Kimberly L. Edwards2, Liben Chen4, Cynthia A. Berlinicke3, M. Joseph Phillips2,5, Eric A. Pierce6, Jiang Qian3, ✉ ✉ Tza-Huei Wang4,7, David M. Gamm2,5,8 & Donald J. Zack 1,3,9,10 1234567890():,; Photoreceptor loss is a leading cause of blindness, but mechanisms underlying photoreceptor degeneration are not well understood. Treatment strategies would benefit from improved understanding of gene-expression patterns directing photoreceptor development, as many genes are implicated in both development and degeneration. Neural retina leucine zipper (NRL) is critical for rod photoreceptor genesis and degeneration, with NRL mutations known to cause enhanced S-cone syndrome and retinitis pigmentosa. While murine Nrl loss has been characterized, studies of human NRL can identify important insights for human retinal development and disease. We utilized iPSC organoid models of retinal development to molecularly define developmental alterations in a human model of NRL loss. Consistent with the function of NRL in rod fate specification, human retinal organoids lacking NRL develop S- opsin dominant photoreceptor populations. We report generation of two distinct S-opsin expressing populations in NRL null retinal organoids and identify MEF2C as a candidate regulator of cone development. 1 Institute of Genetic Medicine, Johns Hopkins University School of Medicine, Baltimore, USA. 2 Waisman Center, University of Wisconsin-Madison, Madison, USA. 3 Department of Ophthalmology, Wilmer Eye Institute, Johns Hopkins University School of Medicine, Baltimore, USA. -
Gateways to the FANTOM5 Promoter Level Mammalian Expression Atlas
Lizio et al. Genome Biology (2015) 16:22 DOI 10.1186/s13059-014-0560-6 SOFTWARE Open Access Gateways to the FANTOM5 promoter level mammalian expression atlas Marina Lizio1,2, Jayson Harshbarger1,2, Hisashi Shimoji1,2, Jessica Severin1,2, Takeya Kasukawa2, Serkan Sahin1,2, Imad Abugessaisa2, Shiro Fukuda1, Fumi Hori1,2, Sachi Ishikawa-Kato1,2, Christopher J Mungall5, Erik Arner1,2, J Kenneth Baillie7, Nicolas Bertin1,2,19, Hidemasa Bono10, Michiel de Hoon1,2, Alexander D Diehl13, Emmanuel Dimont12, Tom C Freeman7, Kaori Fujieda10, Winston Hide12,17, Rajaram Kaliyaperumal8, Toshiaki Katayama15, Timo Lassmann1,2,18, Terrence F Meehan6, Koro Nishikata16, Hiromasa Ono10, Michael Rehli9, Albin Sandelin11, Erik A Schultes8,14, Peter AC ‘t Hoen8, Zuotian Tatum8, Mark Thompson8, Tetsuro Toyoda16, Derek W Wright7, Carsten O Daub1, Masayoshi Itoh1,2,3, Piero Carninci1,2, Yoshihide Hayashizaki1,3, Alistair RR Forrest1,2*, Hideya Kawaji1,2,3,4* and the FANTOM consortium Abstract The FANTOM5 project investigates transcription initiation activities in more than 1,000 human and mouse primary cells, cell lines and tissues using CAGE. Based on manual curation of sample information and development of an ontology for sample classification, we assemble the resulting data into a centralized data resource (http://fantom. gsc.riken.jp/5/). This resource contains web-based tools and data-access points for the research community to search and extract data related to samples, genes, promoter activities, transcription factors and enhancers across the FANTOM5 atlas. Introduction ontologies [10]). However, the ability of these surveys to One of the most comprehensive ways to study the mo- quantify RNA abundance was limited mainly due to se- lecular basis of cellular function is to quantify the pres- quencing performance. -
743914V1.Full.Pdf
bioRxiv preprint doi: https://doi.org/10.1101/743914; this version posted August 24, 2019. The copyright holder for this preprint (which was not certified by peer review) is the author/funder. All rights reserved. No reuse allowed without permission. 1 Cross-talks of glycosylphosphatidylinositol biosynthesis with glycosphingolipid biosynthesis 2 and ER-associated degradation 3 4 Yicheng Wang1,2, Yusuke Maeda1, Yishi Liu3, Yoko Takada2, Akinori Ninomiya1, Tetsuya 5 Hirata1,2,4, Morihisa Fujita3, Yoshiko Murakami1,2, Taroh Kinoshita1,2,* 6 7 1Research Institute for Microbial Diseases, Osaka University, Suita, Osaka 565-0871, Japan 8 2WPI Immunology Frontier Research Center, Osaka University, Suita, Osaka 565-0871, 9 Japan 10 3Key Laboratory of Carbohydrate Chemistry and Biotechnology, Ministry of Education, 11 School of Biotechnology, Jiangnan University, Wuxi, Jiangsu 214122, China 12 4Current address: Center for Highly Advanced Integration of Nano and Life Sciences (G- 13 CHAIN), Gifu University, 1-1 Yanagido, Gifu-City, Gifu 501-1193, Japan 14 15 *Correspondence and requests for materials should be addressed to T.K. (email: 16 [email protected]) 17 18 19 Glycosylphosphatidylinositol (GPI)-anchored proteins and glycosphingolipids interact with 20 each other in the mammalian plasma membranes, forming dynamic microdomains. How their 21 interaction starts in the cells has been unclear. Here, based on a genome-wide CRISPR-Cas9 22 genetic screen for genes required for GPI side-chain modification by galactose in the Golgi 23 apparatus, we report that b1,3-galactosyltransferase 4 (B3GALT4), also called GM1 24 ganglioside synthase, additionally functions in transferring galactose to the N- 25 acetylgalactosamine side-chain of GPI. -
Protease Effects on the Structure of Acetylcholine Receptor Membranes from Torpedo Californica
PROTEASE EFFECTS ON THE STRUCTURE OF ACETYLCHOLINE RECEPTOR MEMBRANES FROM TORPEDO CALIFORNICA MICHAEL W. KLYMKOWSKY, JOHN E . HEUSER, and ROBERT M. STROUD From the Department of Biochemistry & Biophysics, University of California at San Francisco, San Francisco, California 94143 . Dr . Klymkowsky's present address is MRC Neuroimmunology Project, Department of Zoology, University College London, London WC IE, 6BT, England ABSTRACT Protease digestion of acetylcholine receptor-rich membranes derived from Torpedo californica electroplaques by homogenization and isopycnic centrifugation results in degradation of all receptor subunits without any significant effect on the appearance in electron micrographs, the toxin binding ability, or the sedimentation value of the receptor molecule . Such treatment does produce dramatic changes in the morphology of the normally 0.5- to 2-lm-diameter spherical vesicles when observed by either negative-stain or freeze-fracture electron microscopy . Removal of peripheral, apparently nonreceptor polypeptides by alkali stripping (Neubig et al ., 1979, Proc. Natl. Acad. Sci. U. S. A. 76:690-694) results in increased sensitivity of the acetylcholine receptor membranes to the protease trypsin as indicated by SDS gel electrophoretic patterns and by the extent of morphologic change observed in vesicle structure . Trypsin digestion of alkali-stripped receptor membranes results in a limit degradation pattern of all four receptor subunits, whereupon all the vesicles undergo the morphological transformation to minivesicles -
Interplay Between Gating and Block of Ligand-Gated Ion Channels
brain sciences Review Interplay between Gating and Block of Ligand-Gated Ion Channels Matthew B. Phillips 1,2, Aparna Nigam 1 and Jon W. Johnson 1,2,* 1 Department of Neuroscience, University of Pittsburgh, Pittsburgh, PA 15260, USA; [email protected] (M.B.P.); [email protected] (A.N.) 2 Center for Neuroscience, University of Pittsburgh, Pittsburgh, PA 15260, USA * Correspondence: [email protected]; Tel.: +1-(412)-624-4295 Received: 27 October 2020; Accepted: 26 November 2020; Published: 1 December 2020 Abstract: Drugs that inhibit ion channel function by binding in the channel and preventing current flow, known as channel blockers, can be used as powerful tools for analysis of channel properties. Channel blockers are used to probe both the sophisticated structure and basic biophysical properties of ion channels. Gating, the mechanism that controls the opening and closing of ion channels, can be profoundly influenced by channel blocking drugs. Channel block and gating are reciprocally connected; gating controls access of channel blockers to their binding sites, and channel-blocking drugs can have profound and diverse effects on the rates of gating transitions and on the stability of channel open and closed states. This review synthesizes knowledge of the inherent intertwining of block and gating of excitatory ligand-gated ion channels, with a focus on the utility of channel blockers as analytic probes of ionotropic glutamate receptor channel function. Keywords: ligand-gated ion channel; channel block; channel gating; nicotinic acetylcholine receptor; ionotropic glutamate receptor; AMPA receptor; kainate receptor; NMDA receptor 1. Introduction Neuronal information processing depends on the distribution and properties of the ion channels found in neuronal membranes. -
Evaluation of Variability in Human Protein X-Ray Structures Risa Anzai
bioRxiv preprint doi: https://doi.org/10.1101/202028; this version posted October 12, 2017. The copyright holder for this preprint (which was not certified by peer review) is the author/funder, who has granted bioRxiv a license to display the preprint in perpetuity. It is made available under aCC-BY-NC-ND 4.0 International license. Evaluation of variability in human protein X-ray structures Risa Anzai, Yoshiki Asami, Waka Inoue, Hina Ueno, Koya Yamada, Tetsuji Okada Department of Life Science, Gakushuin University, 1-5-1 Mejiro, Toshima-ku, Tokyo 171-8588, Japan. Corresponding author: Tetsuji Okada, Department of Life Science, Gakushuin University, 1-5-1 Mejiro, Toshima-ku, Tokyo 171-8588, Japan. +81-3-3986-0221, [email protected] Abstract Systematic analysis of statistical and dynamical properties of proteins is critical to understanding cellular events. Extraction of biologically relevant information from a set of X-ray structures is important because it can provide mechanistic details behind the functional properties of protein families, enabling rational comparison between families. Most of the current structure comparisons are pairwise-based, which hampers the global analysis of increasing contents in the Protein Data Bank. Additionally, pairing of protein structures introduces uncertainty with respect to reproducibility because it frequently accompanies other settings such as alignment and/or superimposition. This study introduces intramolecular distance scoring, for the analysis of human proteins, for each of which at least several X-ray structures are available. We show that the results are comprehensively used to overview advances at the atomic level exploration of each protein and protein family. -
RNA Editing at Baseline and Following Endoplasmic Reticulum Stress
RNA Editing at Baseline and Following Endoplasmic Reticulum Stress By Allison Leigh Richards A dissertation submitted in partial fulfillment of the requirements for the degree of Doctor of Philosophy (Human Genetics) in The University of Michigan 2015 Doctoral Committee: Professor Vivian G. Cheung, Chair Assistant Professor Santhi K. Ganesh Professor David Ginsburg Professor Daniel J. Klionsky Dedication To my father, mother, and Matt without whom I would never have made it ii Acknowledgements Thank you first and foremost to my dissertation mentor, Dr. Vivian Cheung. I have learned so much from you over the past several years including presentation skills such as never sighing and never saying “as you can see…” You have taught me how to think outside the box and how to create and explain my story to others. I would not be where I am today without your help and guidance. Thank you to the members of my dissertation committee (Drs. Santhi Ganesh, David Ginsburg and Daniel Klionsky) for all of your advice and support. I would also like to thank the entire Human Genetics Program, and especially JoAnn Sekiguchi and Karen Grahl, for welcoming me to the University of Michigan and making my transition so much easier. Thank you to Michael Boehnke and the Genome Science Training Program for supporting my work. A very special thank you to all of the members of the Cheung lab, past and present. Thank you to Xiaorong Wang for all of your help from the bench to advice on my career. Thank you to Zhengwei Zhu who has helped me immensely throughout my thesis even through my panic.