Heteromeric Transposase Elements Target Insertions Into Specific
Total Page:16
File Type:pdf, Size:1020Kb
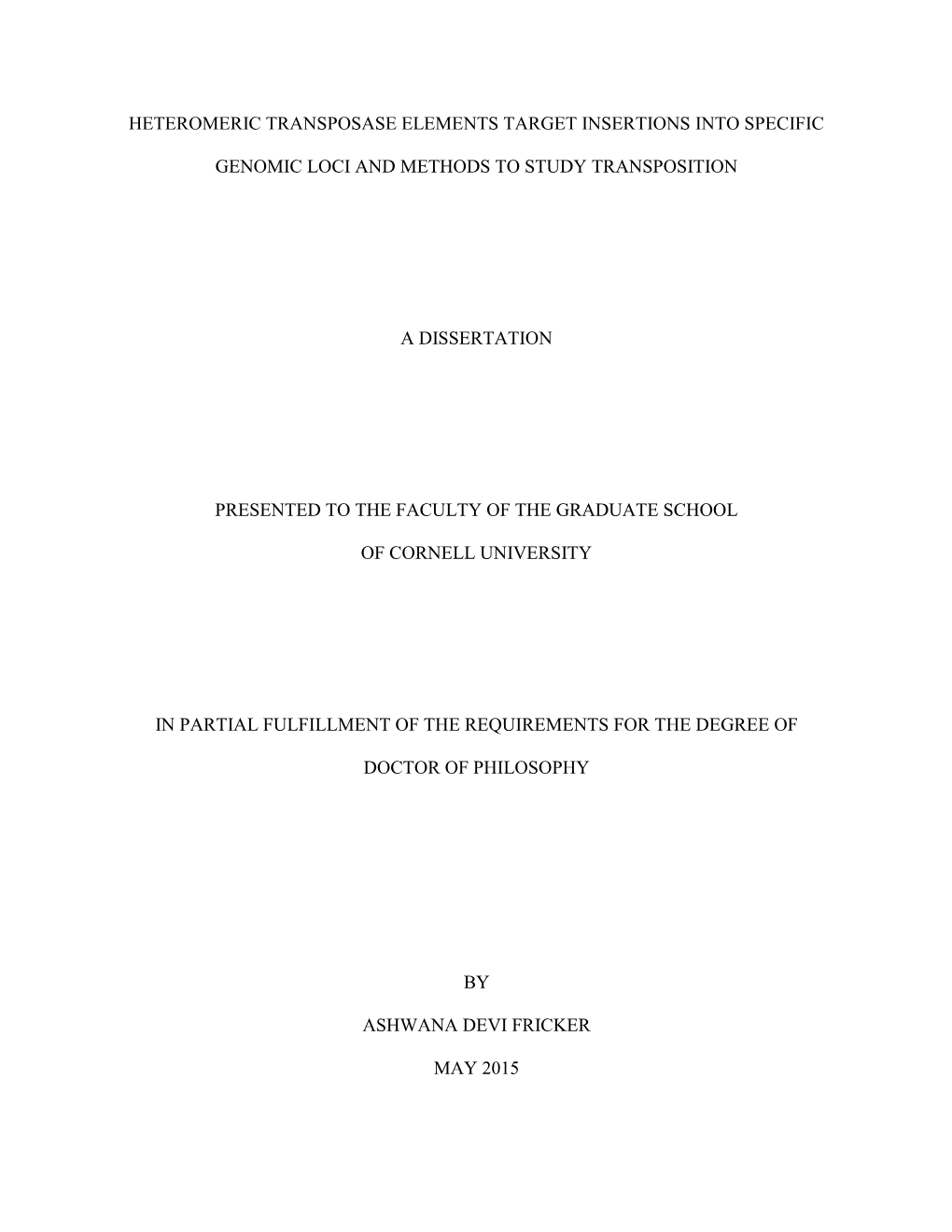
Load more
Recommended publications
-
Specific Binding of Transposase to Terminal Inverted Repeats Of
Proc. Natl. Acad. Sci. USA Vol. 84, pp. 8220-8224, December 1987 Biochemistry Specific binding of transposase to terminal inverted repeats of transposable element Tn3 (transposon/DNA binding protein/DNase I "footprinting") HITOSHI ICHIKAWA*, KAORU IKEDA*, WILLIAM L. WISHARTt, AND EIICHI OHTSUBO*t *Institute of Applied Microbiology, University of Tokyo, Bunkyo-ku, Yayoi 1-1-1, Tokyo 113, Japan; and tDepartment of Molecular Biology, Princeton University, Princeton, NJ 08544 Communicated by Norman Davidson, July 27, 1987 ABSTRACT Tn3 transposase, which is required for trans- containing the Tn3 terminal regions when 8 mM ATP is position of Tn3, has been purified by a low-ionic-strength- present (14). precipitation method. Using a nitrocellulose filter binding In this paper, we study the interaction of Tn3 transposase, assay, we have shown that transposase binds to any restriction which has been purified by a low-ionic-strength-precipitation fragment. However, binding of the transposase to specific method, with DNA. We demonstrate that the purified fragments containing the terminal inverted repeat sequences of transposase is an ATP-independent DNA binding protein, Tn3 can be demonstrated by treatment of transposase-DNA which has interesting properties that may be involved in the complexes with heparin, which effectively removes the actual transposition event of Tn3. transposase bound to the other nonspecific fragments at pH 5-6. DNase I "footprinting" analysis showed that the MATERIALS AND METHODS transposase protects an inner 25-base-pair region of the 38- base-pair terminal inverted repeat sequence of Tn3. This Bacterial Strains and Plasmids. The bacterial strains used protection is not dependent on pH. -
A Sleeping Beauty Mutagenesis Screen Reveals a Tumor Suppressor Role for Ncoa2/Src-2 in Liver Cancer
A Sleeping Beauty mutagenesis screen reveals a tumor suppressor role for Ncoa2/Src-2 in liver cancer Kathryn A. O’Donnella,b,1,2, Vincent W. Kengc,d,e, Brian Yorkf, Erin L. Reinekef, Daekwan Seog, Danhua Fanc,h, Kevin A. T. Silversteinc,h, Christina T. Schruma,b, Wei Rose Xiea,b,3, Loris Mularonii,j, Sarah J. Wheelani,j, Michael S. Torbensonk, Bert W. O’Malleyf, David A. Largaespadac,d,e, and Jef D. Boekea,b,i,2 Departments of aMolecular Biology and Genetics, iOncology, jDivision of Biostatistics and Bioinformatics, and kPathology, and bThe High Throughput Biology Center, The Johns Hopkins University School of Medicine, Baltimore, MD 21205; cMasonic Cancer Center, dDepartment of Genetics, Cell Biology, and Development, eCenter for Genome Engineering, and hBiostatistics and Bioinformatics Core, University of Minnesota, Minneapolis, MN 55455; fDepartment of Molecular and Cellular Biology, Baylor College of Medicine, Houston, TX 77030; and gLaboratory of Experimental Carcinogenesis, Center for Cancer Research, National Cancer Institute, National Institutes of Health, Bethesda, MD 20892 AUTHOR SUMMARY Emerging data from cancer ge- functions are altered. This ap- fi nome-sequencing studies have Sleeping Beauty (SB) transposase proach identi ed at least 16 demonstrated that human genes/loci that contribute to liver tumors exhibit tremendous Transposon array with gene trap (GT) tumor development. complexity and heterogeneity in We next validated that the the number and nature of iden- genes identified in the SB screen tified mutations (1). Based on contribute to tumor initiation these findings, there is an in- SB mobilization and/or progression using in vitro creasing need for in vivo vali- and in vivo cancer model sys- dation of genes whose altered tems. -
RNA As a Source of Transposase for Sleeping Beauty-Mediated Gene Insertion and Expression in Somatic Cells and Tissues
doi:10.1016/j.ymthe.2005.10.014 SHORT COMMUNICATION RNA as a Source of Transposase for Sleeping Beauty-Mediated Gene Insertion and Expression in Somatic Cells and Tissues Andrew Wilber,1 Joel L. Frandsen,1 Jennifer L. Geurts,1 David A. Largaespada,1 Perry B. Hackett,1,2 and R. Scott McIvor1,* 1The Arnold and Mabel Beckman Center for Transposon Research, Gene Therapy Program, Institute of Human Genetics, and Department of Genetics, Cell Biology and Development, University of Minnesota, Minneapolis, MN 55455, USA 2Discovery Genomics, Inc., Minneapolis, MN 55455, USA *To whom correspondence and reprint requests should be addressed at The Department of Genetics, Cell Biology, and Development, 6-160 Jackson Hall, 321 Church Street SE, University of Minnesota, Minneapolis, MN 55455, USA. Fax: +1 612 625 9810. E-mail: [email protected]. Available online 20 December 2005 Sleeping Beauty (SB) is a DNA transposon capable of mediating gene insertion and long-term expression in vertebrate cells when co-delivered with a source of transposase. In all previous reports of SB-mediated gene insertion in somatic cells, the transposase component has been provided by expression of a co-delivered DNA molecule that has the potential for integration into the host cell genome. Integration and continued expression of a gene encoding SB transposase could be problematic if it led to transposon re-mobilization and re-integration. We addressed this potential problem by supplying the transposase-encoding molecule in the form of mRNA. We show that transposase-encoding mRNA can effectively mediate transposition in vitro in HT1080 cells and in vivo in mouse liver following co-delivery with a recoverable transposon or with a luciferase transposon. -
Licensing RNA-Guided Genome Defense
TIBS-1023; No. of Pages 10 Review Recognizing the enemy within: licensing RNA-guided genome defense Phillip A. Dumesic and Hiten D. Madhani Department of Biochemistry and Biophysics, University of California, San Francisco, CA 94158, USA How do cells distinguish normal genes from transpo- RNAi-related RNA silencing pathways are deeply con- sons? Although much has been learned about RNAi- served genome defense mechanisms that act in organisms related RNA silencing pathways responsible for genome from protist to human to recognize and suppress transpo- defense, this fundamental question remains. The litera- sons [8]. In all of these pathways, 20–30 nucleotide small ture points to several classes of mechanisms. In some RNAs act in complex with Argonaute family proteins to cases, double-stranded RNA (dsRNA) structures pro- silence complementary transcripts. Depending on the duced by transposon inverted repeats or antisense inte- pathway and context, silencing proceeds by a variety of gration trigger endogenous small interfering RNA mechanisms, which include RNA degradation, translation- (siRNA) biogenesis. In other instances, DNA features al repression, and the establishment of repressive histone associated with transposons – such as their unusual modifications [9,10]. The pathways also differ in the means copy number, chromosomal arrangement, and/or chro- by which they generate small RNAs. In the canonical RNAi matin environment – license RNA silencing. Finally, re- pathway, RNase-III-type Dicer enzymes convert long cent studies have identified improper transcript dsRNA into small interfering RNA (siRNA). In contrast, processing events, such as stalled pre-mRNA splicing, PIWI-interacting RNA (piRNA) pathways do not require as signals for siRNA production. -
Exploring the Interplay of Telomerase Reverse Transcriptase and Β-Catenin in Hepatocellular Carcinoma
cancers Review Exploring the Interplay of Telomerase Reverse Transcriptase and β-Catenin in Hepatocellular Carcinoma Srishti Kotiyal and Kimberley Jane Evason * Department of Oncological Sciences, Department of Pathology, and Huntsman Cancer Institute, University of Utah, Salt Lake City, UT 84112, USA; [email protected] * Correspondence: [email protected]; Tel.: +1-801-587-4606 Simple Summary: Liver cancer is one of the deadliest human cancers. Two of the most common molecular aberrations in liver cancer are: (1) activating mutations in the gene encoding β-catenin (CTNNB1); and (2) promoter mutations in telomerase reverse transcriptase (TERT). Here, we review recent findings regarding the interplay between TERT and β-catenin in order to better understand their role in liver cancer. Abstract: Hepatocellular carcinoma (HCC) is one of the deadliest human cancers. Activating muta- tions in the telomerase reverse transcriptase (TERT) promoter (TERTp) and CTNNB1 gene encoding β-catenin are widespread in HCC (~50% and ~30%, respectively). TERTp mutations are predicted to increase TERT transcription and telomerase activity. This review focuses on exploring the role of TERT and β-catenin in HCC and the current findings regarding their interplay. TERT can have contradictory effects on tumorigenesis via both its canonical and non-canonical functions. As a critical regulator of proliferation and differentiation in progenitor and stem cells, activated β-catenin drives HCC; however, inhibiting endogenous β-catenin can also have pro-tumor effects. Clinical studies revealed a significant concordance between TERTp and CTNNB1 mutations in HCC. In Citation: Kotiyal, S.; Evason, K.J. stem cells, TERT acts as a co-factor in β-catenin transcriptional complexes driving the expression Exploring the Interplay of Telomerase of WNT/β-catenin target genes, and β-catenin can bind to the TERTp to drive its transcription. -
Catalytic Domain of Plasmid Pad1 Relaxase Trax Defines a Group Of
Catalytic domain of plasmid pAD1 relaxase TraX defines a group of relaxases related to restriction endonucleases María Victoria Franciaa,1, Don B. Clewellb,c, Fernando de la Cruzd, and Gabriel Moncaliánd aServicio de Microbiología, Hospital Universitario Marqués de Valdecilla e Instituto de Formación e Investigación Marqués de Valdecilla, Santander 39008, Spain; bDepartment of Biologic and Materials Sciences, University of Michigan School of Dentistry, Ann Arbor, MI 48109; cDepartment of Microbiology and Immunology, University of Michigan Medical School, Ann Arbor, MI 48109; and dDepartamento de Biología Molecular e Instituto de Biomedicina y Biotecnología de Cantabria, Universidad de Cantabria–Consejo Superior de Investigaciones Científicas–Sociedad para el Desarrollo Regional de Cantabria, Santander 39011, Spain Edited by Roy Curtiss III, Arizona State University, Tempe, AZ, and approved July 9, 2013 (received for review May 30, 2013) Plasmid pAD1 is a 60-kb conjugative element commonly found in known relaxases show two characteristic sequence motifs, motif I clinical isolates of Enterococcus faecalis. The relaxase TraX and the containing the catalytic Tyr residue (which covalently attaches to primary origin of transfer oriT2 are located close to each other and the 5′ end of the cleaved DNA) and motif III with the His-triad have been shown to be essential for conjugation. The oriT2 site essential for relaxase activity (facilitating the cleavage reaction contains a large inverted repeat (where the nic site is located) by activation of the catalytic Tyr). This His-triad has been used as adjacent to a series of short direct repeats. TraX does not show a relaxase diagnostic signature (12). fi any of the typical relaxase sequence motifs but is the prototype of Plasmid pAD1 relaxase, TraX, was identi ed (9) and shown to oriT2 a unique family of relaxases (MOB ). -
Roles of Methylation and Sequestration in the Mechanisms of DNA Replication in Some Members of the Enterobacteriaceae Family
Chapter 12 Roles of Methylation and Sequestration in the Mechanisms of DNA Replication in some Members of the Enterobacteriaceae Family Amine Aloui, Alya El May, Saloua Kouass Sahbani and Ahmed Landoulsi Additional information is available at the end of the chapter http://dx.doi.org/10.5772/51724 1. Introduction When growing cells divide, they need to copy their genetic material and distribute it to en‐ sure that each daughter cell receives one copy. This is a challenging task especially when the enormous length of the DNA compared to the cell size is considered. During DNA replica‐ tion, organization of the chromosomes is even more demanding, since replication forks con‐ tinuously produce new DNA. This DNA contains all the information required to build the cells and tissues of a prokaryotic or an eukaryotic organism. The exact replication of this in‐ formation in any species assures its genetic continuity from generation to generation and is critical to the normal development of an individual. The information stored in DNA is ar‐ ranged in hereditary units known as genes that control the identifiable traits of an organism. Discovery of the structure of DNA and subsequent elucidation of how DNA directs synthe‐ sis of RNA, which then directs assembly of proteins -the so-called central dogma - were monumental achievements that marked the early days of molecular biology. However, the simplified representation of the central dogma as DNA → RNA → protein does not reflect the role of proteins in the synthesis of nucleic acids. Moreover, proteins are largely responsi‐ ble for regulating DNA replication and gene expression, the entire process whereby the in‐ formation encoded in DNA is decoded into the proteins that characterize various cell types. -
The Obscure World of Integrative and Mobilizable Elements Gérard Guédon, Virginie Libante, Charles Coluzzi, Sophie Payot-Lacroix, Nathalie Leblond-Bourget
The obscure world of integrative and mobilizable elements Gérard Guédon, Virginie Libante, Charles Coluzzi, Sophie Payot-Lacroix, Nathalie Leblond-Bourget To cite this version: Gérard Guédon, Virginie Libante, Charles Coluzzi, Sophie Payot-Lacroix, Nathalie Leblond-Bourget. The obscure world of integrative and mobilizable elements: Highly widespread elements that pirate bacterial conjugative systems. Genes, MDPI, 2017, 8 (11), pp.337. 10.3390/genes8110337. hal- 01686871 HAL Id: hal-01686871 https://hal.archives-ouvertes.fr/hal-01686871 Submitted on 26 May 2020 HAL is a multi-disciplinary open access L’archive ouverte pluridisciplinaire HAL, est archive for the deposit and dissemination of sci- destinée au dépôt et à la diffusion de documents entific research documents, whether they are pub- scientifiques de niveau recherche, publiés ou non, lished or not. The documents may come from émanant des établissements d’enseignement et de teaching and research institutions in France or recherche français ou étrangers, des laboratoires abroad, or from public or private research centers. publics ou privés. Distributed under a Creative Commons Attribution| 4.0 International License G C A T T A C G G C A T genes Review The Obscure World of Integrative and Mobilizable Elements, Highly Widespread Elements that Pirate Bacterial Conjugative Systems Gérard Guédon *, Virginie Libante, Charles Coluzzi, Sophie Payot and Nathalie Leblond-Bourget * ID DynAMic, Université de Lorraine, INRA, 54506 Vandœuvre-lès-Nancy, France; [email protected] (V.L.); [email protected] (C.C.); [email protected] (S.P.) * Correspondence: [email protected] (G.G.); [email protected] (N.L.-B.); Tel.: +33-037-274-5142 (G.G.); +33-037-274-5146 (N.L.-B.) Received: 12 October 2017; Accepted: 15 November 2017; Published: 22 November 2017 Abstract: Conjugation is a key mechanism of bacterial evolution that involves mobile genetic elements. -
Virus World As an Evolutionary Network of Viruses and Capsidless Selfish Elements
Virus World as an Evolutionary Network of Viruses and Capsidless Selfish Elements Koonin, E. V., & Dolja, V. V. (2014). Virus World as an Evolutionary Network of Viruses and Capsidless Selfish Elements. Microbiology and Molecular Biology Reviews, 78(2), 278-303. doi:10.1128/MMBR.00049-13 10.1128/MMBR.00049-13 American Society for Microbiology Version of Record http://cdss.library.oregonstate.edu/sa-termsofuse Virus World as an Evolutionary Network of Viruses and Capsidless Selfish Elements Eugene V. Koonin,a Valerian V. Doljab National Center for Biotechnology Information, National Library of Medicine, Bethesda, Maryland, USAa; Department of Botany and Plant Pathology and Center for Genome Research and Biocomputing, Oregon State University, Corvallis, Oregon, USAb Downloaded from SUMMARY ..................................................................................................................................................278 INTRODUCTION ............................................................................................................................................278 PREVALENCE OF REPLICATION SYSTEM COMPONENTS COMPARED TO CAPSID PROTEINS AMONG VIRUS HALLMARK GENES.......................279 CLASSIFICATION OF VIRUSES BY REPLICATION-EXPRESSION STRATEGY: TYPICAL VIRUSES AND CAPSIDLESS FORMS ................................279 EVOLUTIONARY RELATIONSHIPS BETWEEN VIRUSES AND CAPSIDLESS VIRUS-LIKE GENETIC ELEMENTS ..............................................280 Capsidless Derivatives of Positive-Strand RNA Viruses....................................................................................................280 -
The Bacterial Conjugation Protein Trwb Resembles Ring Helicases And
letters to nature metabolically labelled with BrdU (10 mM, 4 h), trypsinized, and ®xed with 70% ethanol. 17. Stampfer, M. R. et al. Gradual phenotypic conversion associated with immortalization of cultured Nuclei were isolated and stained with propidium iodide and FITC-conjugated anti-BrdU human mammary epithelial cells. Mol. Biol. Cell 8, 2391±2405 (1997). antibodies (Becton Dickinson, USA), as described7. Flow cytometry was performed on a 18. Karlseder, J., Broccoli, D., Dai, Y., Hardy, S. & de Lange, T. p53- and ATM-dependent apoptosis FACS Sorter (Becton Dickinson). All analysed events were gated to remove debris and induced by telomeres lacking TRF2. Science 283, 1321±1325 (1999). aggregates. 19. Artandi, S. E. et al. Telomere dysfunction promotes non-reciprocal translocations and epithelial cancers in mice. Nature 406, 641±645 (2000). Cell death assays 20. Chin, L. et al. p53 De®ciency rescues the adverse effects of telomere loss and cooperates with telomere dysfunction to accelerate carcinogenesis. Cell 97, 527±538 (1999). TUNEL assay for DNA fragmentation was done using an In Situ Cell Death Detection kit 21. Alcorta, D. A. et al. Involvement of the cyclin-dependent kinase inhibitor p16 (INK4A) in replicative (BMB), according to manufacturer's protocol. Alternatively, cells were stained with senescence of normal human ®broblasts. Proc. Natl Acad. Sci. USA 92, 13742±13747 (1996). Annexin-V-FLUOR (BMB) and propidium iodide, and analysed by ¯uorescence 22. Hara, E. et al. Regulation of p16CDKN2 expression and its implications for cell immortalization and microscopy. senescence. Mol. Cell. Biol. 16, 859±867 (1996). 23. Burbano, R. R. et al. -
Sequence-Specific DNA Nicking Endonucleases
BioMol Concepts 2015; 6(4): 253–267 Review Open Access Shuang-yong Xu* Sequence-specific DNA nicking endonucleases DOI 10.1515/bmc-2015-0016 Received May 20, 2015; accepted June 24, 2015 Introduction In this article, I will discuss natural DNA nicking endo- nucleases (NEases or nickases) with 3- to 7-bp specificities, Abstract: A group of small HNH nicking endonucleases e.g. Nt.CviPII (↓CCD, the down arrow indicates the nicked (NEases) was discovered recently from phage or prophage strand as shown) originally found in chlorella virus (1), genomes that nick double-stranded DNA sites ranging engineered NEases such as Nt.BspQI (GCTCTTCN↓), and from 3 to 5 bp in the presence of Mg2+ or Mn2+. The cosN site Nt.BbvCI (CC↓TCAGC) engineered from BspQI and BbvCI of phage HK97 contains a gp74 nicking site AC↑CGC, which restriction endonucleases (REases) (2, 3). The other group is similar to AC↑CGR (R = A/G) of N.φGamma encoded by of DNA NEases contains natural or engineered enzymes Bacillus phage Gamma. A minimal nicking domain of 76 with more than 8-bp target sites, which includes group I amino acid residues from N.φGamma could be fused to intron-encoded homing endonucleases (HEs) (4, 5), other DNA binding partners to generate chimeric NEases engineered nicking variants from LAGLIDAG HEs (6–8), with new specificities. The biological roles of a few small engineered TALE nucleases (TALENs) by fusion of tran- HNH endonucleases (HNHE, gp74 of HK97, gp37 of φSLT, scription activator-like effector (TALE) repeat domain with φ12 HNHE) have been demonstrated in phage and patho- FokI nuclease domain or a MutH nicking variant (9–11), ZF genicity island DNA packaging. -
Negative Control of Replication Initiation by a Novel Chromosomal Locus Exhibiting Exceptional Affinity for Escherichia Coli Dnaa Protein
Downloaded from genesdev.cshlp.org on October 2, 2021 - Published by Cold Spring Harbor Laboratory Press Negative control of replication initiation by a novel chromosomal locus exhibiting exceptional affinity for Escherichia coli DnaA protein Risa Kitagawa,1,3 Toru Ozaki,1 Shigeki Moriya,2 and Tohru Ogawa1,4 1Division of Biological Science, Graduate School of Science, Nagoya University Chikusa-ku, Nagoya 464-8602, Japan; 2Department of Cell Biology, Graduate School of Biological Sciences, Nara Institute of Science and Technology, Takayama-cho, Ikoma 630-0101, Japan Replication of the Escherichia coli chromosome is initiated at a unique site, oriC. Concurrent initiation occurs at all oriC sites present in a cell once, and only once, per cell cycle. A mechanism to ensure cyclic initiation events was found operating through the chromosomal site, datA, a 1-kb segment located at 94.7 min on the genetic map that titrates exceptionally large amounts of the bacterial initiator protein, DnaA. A strain lacking datA grew normally but exhibited an asynchronous initiation phenotype as a result of extra initiation events. This mutant phenotype was suppressed by DnaA-titrating plasmids. Furthermore, mutations in a 9-bp DnaA-binding sequence (the DnaA box) in datA were enough to induce the mutant phenotype. Thus, datA is a novel chromosomal element that appears to adjust a balance between free and bound DnaA for a single initiation event at a fixed time in the bacterial cell cycle. Titration of DnaA to newly duplicated datA during oriC sequestration, which is mediated by hemimethylated GATC sequences in oriC and the SeqA protein, would contribute to prevention of reinitiations when oriC is desequestered.