Quantification and Rationalization of the Higher Affinity of Sodium Over Potassium to Protein Surfaces
Total Page:16
File Type:pdf, Size:1020Kb
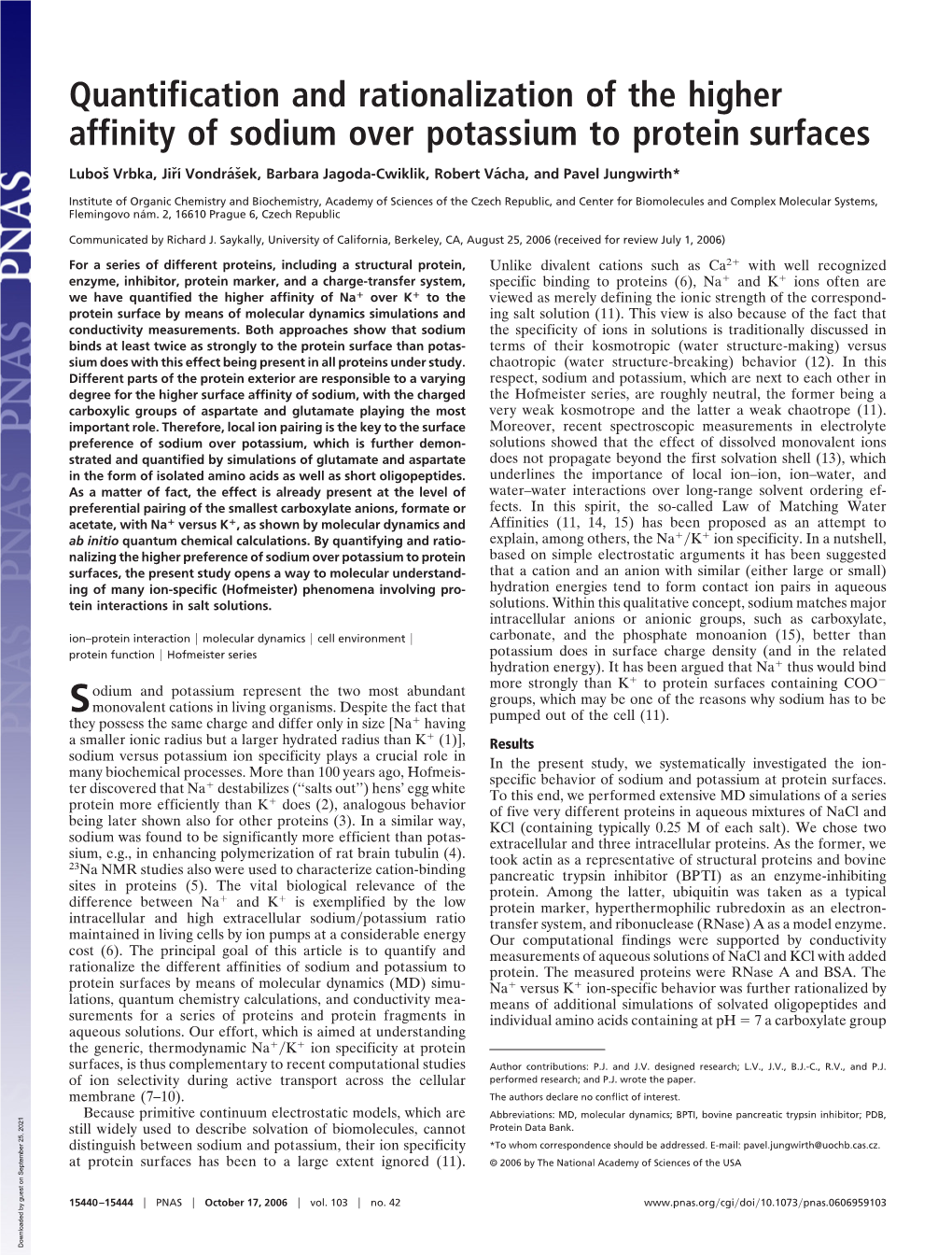
Load more
Recommended publications
-
Is Lithium Brine Water? (Desalination)
Desalination xxx (xxxx) xxx Contents lists available at ScienceDirect Desalination journal homepage: www.elsevier.com/locate/desal Is lithium brine water? Mojtaba Ejeian a, Alexander Grant b, Ho Kyong Shon c, Amir Razmjou c,* a Institute of Refrigeration and Cryogenics, Engineering Research Center of Solar Energy (MOE China), Shanghai Jiao Tong University, 800 Dongchuan Road, Shanghai 200240, China b Principal, Jade Cove Partners, San Francisco, California, United States of America c Centre for Technology in Water and Wastewater, University of Technology Sydney, New South Wales, Australia HIGHLIGHTS • The rapid expansion of applications of Lithium-ion batteries has raised Li demand. • Overexploitation of Li brine resources such as Salar de Atacama raised concerns. • There is an ongoing debate over the definition of Lithium brine as water or minerals. • The debate has frustrated basic measures for moving towards a sustainable operation. • Here, we used a molecular level approach to prove that the brine is a type of water. ARTICLE INFO ABSTRACT Keywords: With the development of light and rechargeable batteries for electric vehicles, global demand for lithium has Lithium increased considerably in recent years. This has drawn more attention to how lithium is produced, especially on Brine primary extraction operations such as those at the Salar de Atacama in Northern Chile. There are concerns that Lithium Ion Batteries brine extraction at the Atacama could irreversibly damage the basin's complex hydrological system. However, Electric Vehicles differing opinions over the definition of water have frustrated basic action measures for minimizing impacts of Hydrogeology Resource Extraction operations like these. Some lithium industry stakeholders have historically described brine as a mineral, while Molecular Dynamics others emphasize that brine is also a type of water in a complex network of different water resources. -
The S-Block Elements of the Periodic Table Are Those in Which the Last Electron Enters the Outermost S-Orbital
Chapter-10 The s-block elements The s-block elements: The s-block elements of the Periodic Table are those in which the last electron enters the outermost s-orbital. The s-orbital can accommodate only two electrons, two groups (1 & 2) belong to the s- block of the Periodic Table. Group 1 elements: alkali metals Lithium Li Sodium Na Potassium K Rubidium Rb Caesium Cs Francium. Fr Why they are known as alkali metals? They are collectively known as the alkali metals. These are so called because they form hydroxides on reaction with water which are strongly alkaline in nature. Electronic Configuration The general electronic configuration of alkali elements is [noble gas] ns1 Lithium Li 1s22s1 or [He]2s1 Sodium Na 1s22s22p63s1or [Ne] 3s1 Potassium K 1s22s22p63s23p64s1 or [Ar]4s1 Rubidium Rb 1s22s22p63s23p63d104s24p65s1or [Kr]5s1 Caesium Cs 1s22s22p63s23p63d104s2 4p64d105s25p66s1 or [Xe] 6s1 Francium. Fr [Rn]7s1 Occurrence: Sodium and potassium are abundant and lithium, rubidium and caesium have much lower abundances. Francium is highly radioactive; its longest-lived isotope 223Fr has a half-life of only 21 minutes. All the alkali metals have one valence electron, ns1 outside the noble gas core. The loosely held s-electron in the outermost valence shell of these elements makes them the most electropositive metals. They readily lose electron to give monovalent M+ ions. Hence,they are never found in free state in nature. Atomic and Ionic Radii The alkali metal atoms have the largest sizes in a particular period of the periodic table. Withincrease in atomic number, the atom becomes larger. The monovalent ions (M+) are smaller than the parent atom. -
Development and Characterization of Thermochemical Materials Based on Salt Hydrates and Salt Alcoholates
Development and characterization of thermochemical materials based on salt hydrates and salt alcoholates Von der Fakultät Nachhaltigkeit der Leuphana Universität Lüneburg zur Erlangung des Grades Doktorin der Naturwissenschaften (Dr. rer. nat.) genehmigte Dissertation von Dipl.-Ing. Kathrin Korhammer geboren am 04.03.1984 in Altötting November 2018 Eingereicht am: 29.11.2018 Mündliche Verteidigung (Disputation) am: 24.02.2020 Erstbetreuer und Erstgutachter: Prof. Dr.-Ing. Wolfgang K. L. Ruck Zweitgutachter: Prof. Dr. Oliver Opel Drittgutachter: Prof. Dr. Frédéric Kuznik Die einzelnen Beiträge des kumulativen Dissertationsvorhabens sind oder werden wie folgt veröffentlicht: I Fopah Lele A, Korhammer K, Wegscheider N, Rammelberg HU, Osterland T, Ruck W. Thermal conductivity measurement of salt hydrate as porous material using calorimetric (DSC) method. 8th World Conference on Experimental Heat Transfer, Fluid Mechanics and Thermodynamics (ExHFT), Lisboa, Portugal: A. Faria - Edicao Electronica Lda.; 2013. II Druske M-M, Fopah-Lele A, Korhammer K, Rammelberg HU, Wegscheider N, Ruck W, et al. Developed materials for thermal energy storage: Synthesis and characterization. Energy Procedia 2014;61:96–9. III Korhammer K, Druske M-M, Fopah-Lele A, Rammelberg HU, Wegscheider N, Opel O, et al. Sorption and thermal characterization of composite materials based on chlorides for thermal energy storage. Applied Energy 2016;162:1462–72. IV Korhammer K, Apel C, Osterland T, Ruck WKL. Reaction of calcium chloride and mag- nesium chloride and their mixed salts with ethanol for thermal energy storage. Energy Procedia 2016;91:161–71. V Korhammer K, Mihály J, Bálint S, Trif L, Vass Á, Tompos A, et al. Reversible formation of alcohol solvates and their potential use for heat storage. -
Application to the Lithium Ionic Electrolyte Systems with Excess Gibbs Energy Model Including Solvation
Application to the Lithium ionic Electrolyte Systems with Excess Gibbs Energy Model including Solvation Sung Bin Park, Chul Soo Lee Dept. of Chemical & Biological Engineering, Korea University Thermodynamics & Properties Lab. Scheme Introduction Theoretical Background Results Conclusion Thermodynamics & Properties Lab. Introduction Aqueous Electrolyte Solution A wide variety of important chemical processes Wastewater treatment, extractive distillation, solution crystallization, desalination, gas scrubbing etc. Previous Models GE Model Debye-Hückel (1923), Bromley (1973), Pitzer (1973) Meissner & Tester (1972), Chen et al. (1982) Primitive Model Wasman et al. (1972), Blum (1975), Harvey et al. (1989), Taghikhani et al. (2000) Nonprimitive Model Planche et al. (1981), Ball et al. (1985), Fürst et al. (1993), Zuo et al. (1998) Acceptable accuracy up to I=6.0 or less Thermodynamics & Properties Lab. Hydration Theory GE model Ghosh and Patwardhan (1990) Based on lithium chrolide as a reference electrolyte Hydration energy, function of the total moles of water hydrated per kg of solution Up to I=24 for 150 electrolyte solutions Schoenert (1990, 1991,1993, 1994) Modified hydration model of Robinson and Stokes Transfer of water to the hydration spheres of ions Up to m=1 for HCl,LiCl,NaCl,KCl,CsCl,NH4Cl,NaBr Thermodynamics & Properties Lab. Hydration Model SAFT Approach Gil-Villegas et al. (2001) Ionic contribution : MSA Solvent-solvent, solvent-ion, ion-ion pairing Applied to the NaCl solution up to 10 m Paricaud et al. (2001) Applied to the NaOH solution up to 22 m In present work Solvation contribution from the Veytsman statistics Explicitly added to Lee et al. model Application to LiCl, LiBr, LiI and LiClO4 solutions Thermodynamics & Properties Lab. -
Investigation Into the Potential of Mgso4 for Interseasonal Domestic Thermochemical Energy Storage
Investigation into the potential of MgSO4 for interseasonal domestic thermochemical energy storage By Daniel Mahon Doctoral Thesis Submitted in partial fulfillment of the requirements for the award of Doctor of Philosophy of Loughborough University June 2018 © by Daniel Mahon 2018 I I. Abstract Approximately 26% of the UK’s primary energy consumption is used specifically for Domestic Space Heating (DSH) and Domestic Hot Water (DHW) production [1]. The majority of this, 88%, comes directly from gas and oil with only 2% coming from renewable energy sources [1]. Decarbonising DSH and DHW represents a huge challenge for the UK’s government which is targeting a reduction of CO2 emissions of 80% by 2050 [2]. The amount of energy utilised from renewable sources can be increased by effective Thermal Energy Storage (TES). In a domestic environment thermal energy is typically required when the energy supplied from renewable sources is low (i.e. thermal energy demand is high in the winter and low in the summer), interseasonal Thermochemical Energy Storage (TCES) offers a solution to this problem. TCES has the ability to store thermal energy from the summer months within chemical bonds and release the stored heat when required with heat losses of only around 15%. 3 Magnesium sulphate heptahydrate (MgSO4.7H2O) has the potential to store 2.8GJ/m of energy, is a low cost, non-toxic, safe material that can be dehydrated to MgSO4.0.1H2O (fully charged) at 150˚C making it suitable for domestic integration. Research has shown that when MgSO4 is used for TCES it suffers from problematic issues such as agglomeration. -
Hydration Energy Alkali Metal Alkaline Earth Metals
2- H2O2 changes Cr2O7 ion to CrO5 in an acidic medium, the oxidation state of Cr in CrO5 is (a) +6 (b) +5 (c) -10 (d) +3 Ques) Write balanced chemical equation for the following reaction: – (i) Permanganate ion (MnO4 ) reacts with sulphur dioxide gas in acidic medium to produce Mn2+ and hydrogensulphate ion. Chandni Prince kumar Khushi jha Chanduchandana Chandana s block elements General properties Configuration & Physical state Alkali Alkaline earth Metal metals ● 1 e- in outermost shell ● 2e- in outermost shell ● General formulas ns1 ● General formula ns2 ● All are silvery white ● All are greyish white ● Light soft, malleable ● These are harder than and ductile metals with alkali metals metallic lustre Atomic size Alkali Alkaline earth Metal metals ● Biggest in their ● Smaller than IA group respective period elements ● Size increases from Li ● Size increases to Fr du to addition of gradually from Be to an extra shell. Ra Li < Na < K < Rb < Cs < Be < Mg < Ca < Sr < Ba Fr Ionization potential Alkali Alkaline earth Metal metals ● First I.P. is very less ● First I.P. is higher than because of bigger IA group because of atomic size and only e- smaller atomic size in outermost shell. and completely filled s- orbital (stability) ● Second I.P. is very ● Second I.P. is lesser high because of than IA group. achieving inert gas configuration ● Order of I.P. ● Order of I.P. Li > Na > K > Rb > Cs Be > Mg > Ca > Sr > Ba (1st and 2nd I.P. (1st and 2nd I.P. difference > 16eV) difference < 11eV) Electropositive or metallic character Alkali Alkaline earth Metal metals ● electropositivity ● Their atomic size is ∝1/ionisation smaller than IA group so these are less ● Due to their larger ● Electropositivity size, electron can increases from Be and easily be removed to Ba form M+ ion. -
Chapter 10 the S-Block Elements
Chemistry (Chapter – 10) (The s – Block Elements) (Class – XI) Question 10.1: What are the common physical and chemical features of alkali metals? Answer 10.1: Physical properties of alkali metals: (1) They are quite soft and can be cut easily. Sodium metal can be easily cut using a knife. (2) They are light coloured and are mostly silvery white in appearance. (3) They have low density because of the large atomic sizes. The density increases down the group from Li to Cs. The only exception to this is K, which has lower density than Na. (4) The metallic bonding present in alkali metals is quite weak. Therefore, they have low melting and boiling points. (5) Alkali metals and their salts impart a characteristic colour to flames. This is because the heat from the flame excites the electron present in the outermost orbital to a high energy level. When this excited electron reverts back to the ground state, it emits excess energy as radiation that falls in the visible region. (6) They also display photoelectric effect. When metals such as Cs and K are irradiated with light, they lose electrons. Chemical properties of alkali metals: Alkali metals are highly reactive due to their low ionization enthalpy. As we move down the group, the reactivity increases. (1) They react with water to form respective oxides or hydroxides. As we move down the group, the reaction becomes more and more spontaneous. (2) They react with water to form their respective hydroxides and dihydrogens. The general reaction for the same is given as (3) They react with dihydrogen to form metal hydrides. -
Effects of Electronegativity and Hydration Energy on the Selective Adsorption of Heavy Metal Ions by Synthetic Nax Zeolite
materials Article Effects of Electronegativity and Hydration Energy on the Selective Adsorption of Heavy Metal Ions by Synthetic NaX Zeolite Xianyuan Fan 1,2, Hong Liu 1,2,*, Emmanuella Anang 1 and Dajun Ren 1,2 1 Department of Resource and Environmental Engineering, Wuhan University of Science and Technology, Wuhan 430081, China; [email protected] (X.F.); [email protected] (E.A.); [email protected] (D.R.) 2 Hubei Key Laboratory for Efficient Utilization and Agglomeration of Metallurgic Mineral Resources, Wuhan University of Science and Technology, Wuhan 430081, China * Correspondence: [email protected]; Tel.: +86-139-7148-8669 Abstract: The adsorption capacity of synthetic NaX zeolite for Pb2+, Cd2+, Cu2+ and Zn2+ in single and multi-component systems were investigated. The effects of electronegativity and hydration energy on the selective adsorption, as well as potential selective adsorption mechanism of the NaX zeolite for Pb2+, Cd2+, Cu2+ and Zn2+ were also discussed. The maximum adsorption capacity order of the heavy metals in the single system was Pb2+ > Cd2+ > Cu2+ > Zn2+, and this could be related to their hydration energy and electronegativity. The values of the separation factors (α) and affinity 2+ 2+ constant (KEL) in different binary systems indicated that Pb was preferentially adsorbed, and Zn presented the lowest affinity for NaX zeolite. The selective adsorption capacities of the metals were in 2+ 2+ 2+ 2+ Citation: Fan, X.; Liu, H.; Anang, E.; the order, Pb > Cd ≈ Cu > Zn . The trend for the selective adsorption of NaX zeolite in ternary Ren, D. Effects of Electronegativity and quaternary systems was consistent with that in the binary systems. -
Electrolyte Solutions: Thermodynamics, Crystallization, Separation Methods
Downloaded from orbit.dtu.dk on: Oct 06, 2021 Electrolyte Solutions: Thermodynamics, Crystallization, Separation methods Thomsen, Kaj Link to article, DOI: 10.11581/dtu:00000073 Publication date: 2009 Document Version Publisher's PDF, also known as Version of record Link back to DTU Orbit Citation (APA): Thomsen, K. (2009). Electrolyte Solutions: Thermodynamics, Crystallization, Separation methods. https://doi.org/10.11581/dtu:00000073 General rights Copyright and moral rights for the publications made accessible in the public portal are retained by the authors and/or other copyright owners and it is a condition of accessing publications that users recognise and abide by the legal requirements associated with these rights. Users may download and print one copy of any publication from the public portal for the purpose of private study or research. You may not further distribute the material or use it for any profit-making activity or commercial gain You may freely distribute the URL identifying the publication in the public portal If you believe that this document breaches copyright please contact us providing details, and we will remove access to the work immediately and investigate your claim. Electrolyte Solutions: Thermodynamics, Crystallization, Separation methods 2009 Kaj Thomsen, Associate Professor, DTU Chemical Engineering, Technical University of Denmark [email protected] 1 List of contents 1 INTRODUCTION ................................................................................................................. 5 2 CONCENTRATION -
Hydration and Diffusion of H+, Li+, Na+, Cs+ Ions in Cation-Exchange
membranes Article Hydration and Diffusion of H+, Li+, Na+, Cs+ Ions in Cation-Exchange Membranes Based on Polyethylene- and Sulfonated-Grafted Polystyrene Studied by NMR Technique and Ionic Conductivity Measurements Vitaliy I. Volkov 1,2,*, Alexander V. Chernyak 1,2, Daniil V. Golubenko 3, Vladimir A. Tverskoy 4, Georgiy A. Lochin 1,5, Ervena S. Odjigaeva 1,5 and Andrey B. Yaroslavtsev 3 1 Institute of Problems of Chemical Physics RAS, 142432 Chernogolovka, Russia; [email protected] (A.V.C.); [email protected] (G.A.L.); [email protected] (E.S.O.) 2 Scientific Center in Chernogolovka RAS, 142432 Chernogolovka, Russia 3 Kurnakov Institute of General and Inorganic Chemistry RAS, 119991 Moscow,Russia; [email protected] (D.V.G.); [email protected] (A.B.Y.) 4 Lomonosov Institute of Fine Chemical Technologies, MIREA–Russian Technological University, 119571 Moscow, Russia; [email protected] 5 Faculty of Chemistry, Lomonosov Moscow State University, 119991 Moscow, Russia * Correspondence: [email protected] or [email protected] Received: 12 August 2020; Accepted: 28 September 2020; Published: 1 October 2020 Abstract: The main particularities of sulfonate groups hydration, water molecule and alkaline metal cation translation mobility as well as ionic conductivity were revealed by NMR and impedance spectroscopy techniques. Cation-exchange membranes MSC based on cross-linked sulfonated polystyrene (PS) grafted on polyethylene with ion-exchange capacity of 2.5 mg-eq/g were investigated. Alkaline metal cation hydration numbers (h) calculated from temperature dependences of 1H chemical shift of water molecule for membranes equilibrated with water vapor at RH = 95% are 5, 6, and 4 for Li+, Na+, and Cs+ ions, respectively. -
Sodium Ion Hydration: Coordination Shell Peculiarities Depending On
Flexibility and regularity of the hydration structure of a sodium ion. Nonempirical insight Yulia V. Novakovskaya Moscow State University, Leninskie Gory 3/1, Moscow, 119991 Russia E-mail: [email protected] + Abstract: The stationary nonempirical simulations of Na (H2O)n clusters with n in a range of 28 to 51 carried out at the density functional level with a hybrid B3LYP functional and the Born- Oppenheimer molecular dynamics modeling of the size selected clusters reveal the interrelated structural and energetic peculiarities of sodium hydration structures. Surface, bulk, and transient configurations of the clusters are distinguished with the different location of the sodium nucleus (close to either the spatial center of the structure or one of its side faces) and its consistently changing coordination number (which typically equals five or six). The <rNaO> mean Na-O distances for the first-shell water molecules are found to depend both on the spatial character of the structure and the local coordination of sodium. The <rNaO> values are compared to different experimental estimates, and the virtual discrepancy of the latter is explained based on the results of the cluster simulations. Different coordination neighborhoods of sodium are predicted depending on its local fraction in the actual specimens. Key words: sodium ion hydration; sodium-water clusters; coordination number; radial distribution function; stationary nonempirical simulations; Born-Oppenheimer molecular dynamics; vibrational thermal energy INTRODUCTION Hydration of ions, especially alkaline metal ions which regulate numerous intra-, inter-, and extracellular processes in living organisms and are present in various current sources and electrolyte baths of different purposes, was repeatedly studied for a long time, and it may seem now that everything is already clear. -
Free Energy Calculations of Ion Hydration: an Analysis of the Born Model in Terms of Microscopic Simulations
4320 J. Phys. Chem. 1989, 93, 4320-4327 Free Energy Calculations of Ion Hydration: An Analysis of the Born Model in Terms of Microscopic Simulations B. Jayaram,t Richard Fine,I Kim Sharp,t and Barry Honig*Vt Department of Biochemistry and Molecular Biophysics, Columbia University, New York, New York 10032, and Deparzment of Biological Sciences, Columbia University, New York, New York 10027 (Received: October 4, 1988) The free energy perturbation technique is used in conjunction with Monte Carlo simulations to calculate the electrostatic contribution to the hydration free energy of a hypothetical cation whose charge varies in the range 0-3 au. The results are used to examine the validity of the continuum (Born) treatment of ion hydration. Saturation of the orientational polarizability of the water dipoles begins to become significant at values of the ionic charge of about +0.?5, a considerably higher value than predicted from a Langevin analysis. Moreover, due to the opposing effect of electrostriction on the dielectric response, the continuum prediction of a quadratic charge dependence of the hydration free energy is reproduced for values of the ionic charge up to approximately +1.1. These results suggest that dielectric saturation is insignificant for monovalent cations. Above charges of 1.1 the first solvation shell becomes fully saturated while the remaining water molecules continue to respond quadratically with increasing charge. It is argued that to within a few percent the assumptions of the Born model (without corrections for dielectric saturation) can be justified in terms of microscopic simulations. Introduction defining the cavity formed by an ion.3 Calculated solvation en- An accurate theoretical treatment of the solvation of simple thalpies based on these radii (increased uniformly by ?%) are in ions in water has been difficult to obtain despite the apparent excellent agreement with experiment even for multivalent ions.