(II) Phycoremediation Using Scenedesmus Obliquus: Cell Physicochemical Properties, Biochemical Composition and Metabolomic Pro Ling
Total Page:16
File Type:pdf, Size:1020Kb
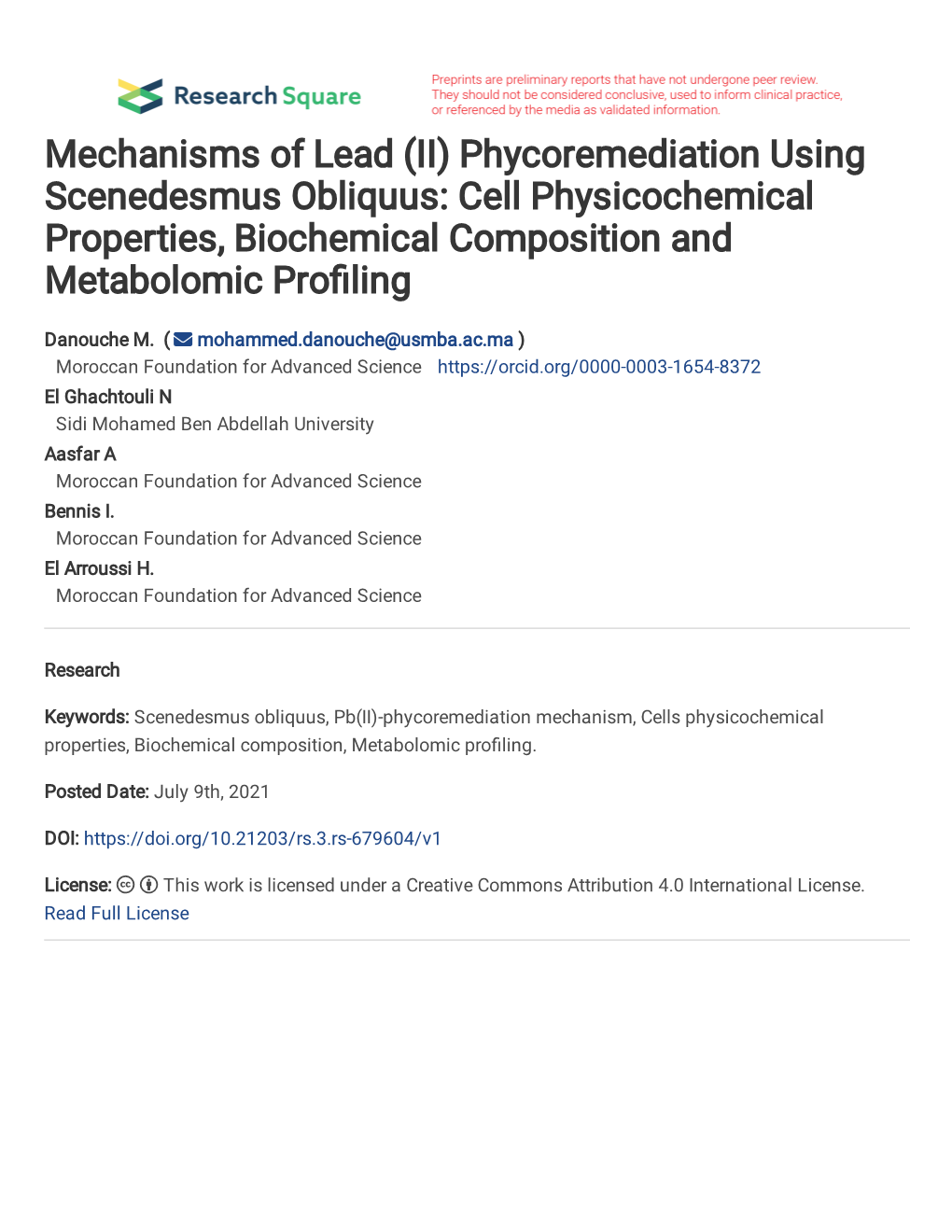
Load more
Recommended publications
-
(12) United States Patent (10) Patent No.: US 9,375.433 B2 Dilly Et Al
US009375433B2 (12) United States Patent (10) Patent No.: US 9,375.433 B2 Dilly et al. (45) Date of Patent: *Jun. 28, 2016 (54) MODULATORS OF ANDROGENSYNTHESIS (52) U.S. Cl. CPC ............. A6 IK3I/519 (2013.01); A61 K3I/201 (71) Applicant: Tangent Reprofiling Limited, London (2013.01); A61 K3I/202 (2013.01); A61 K (GB) 31/454 (2013.01); A61K 45/06 (2013.01) (72) Inventors: Suzanne Dilly, Oxfordshire (GB); (58) Field of Classification Search Gregory Stoloff, London (GB); Paul USPC .................................. 514/258,378,379, 560 Taylor, London (GB) See application file for complete search history. (73) Assignee: Tangent Reprofiling Limited, London (56) References Cited (GB) U.S. PATENT DOCUMENTS (*) Notice: Subject to any disclaimer, the term of this 5,364,866 A * 1 1/1994 Strupczewski.......... CO7C 45/45 patent is extended or adjusted under 35 514,254.04 U.S.C. 154(b) by 0 days. 5,494.908 A * 2/1996 O’Malley ............. CO7D 261/20 514,228.2 This patent is Subject to a terminal dis 5,776,963 A * 7/1998 Strupczewski.......... CO7C 45/45 claimer. 514,217 6,977.271 B1* 12/2005 Ip ........................... A61K 31, 20 (21) Appl. No.: 14/708,052 514,560 OTHER PUBLICATIONS (22) Filed: May 8, 2015 Calabresi and Chabner (Goodman & Gilman's The Pharmacological (65) Prior Publication Data Basis of Therapeutics, 10th ed., 2001).* US 2015/O238491 A1 Aug. 27, 2015 (Cecil's Textbook of Medicine pp. 1060-1074 published 2000).* Stedman's Medical Dictionary (21st Edition, Published 2000).* Okamoto et al (Journal of Pain and Symptom Management vol. -
WO 2017/074902 Al 4 May 20 17 (04.05.2017) W P O P C T
(12) INTERNATIONAL APPLICATION PUBLISHED UNDER THE PATENT COOPERATION TREATY (PCT) (19) World Intellectual Property Organization International Bureau (10) International Publication Number (43) International Publication Date WO 2017/074902 Al 4 May 20 17 (04.05.2017) W P O P C T (51) International Patent Classification: AO, AT, AU, AZ, BA, BB, BG, BH, BN, BR, BW, BY, A61K 8/37 (2006.01) A61Q 19/00 (2006.01) BZ, CA, CH, CL, CN, CO, CR, CU, CZ, DE, DJ, DK, DM, A61K 31/215 (2006.01) DO, DZ, EC, EE, EG, ES, FI, GB, GD, GE, GH, GM, GT, HN, HR, HU, ID, IL, IN, IR, IS, JP, KE, KG, KN, KP, KR, (21) International Application Number: KW, KZ, LA, LC, LK, LR, LS, LU, LY, MA, MD, ME, PCT/US2016/058591 MG, MK, MN, MW, MX, MY, MZ, NA, NG, NI, NO, NZ, (22) International Filing Date: OM, PA, PE, PG, PH, PL, PT, QA, RO, RS, RU, RW, SA, 25 October 2016 (25.10.201 6) SC, SD, SE, SG, SK, SL, SM, ST, SV, SY, TH, TJ, TM, TN, TR, TT, TZ, UA, UG, US, UZ, VC, VN, ZA, ZM, (25) Filing Language: English ZW. (26) Publication Language: English (84) Designated States (unless otherwise indicated, for every (30) Priority Data: kind of regional protection available): ARIPO (BW, GH, 62/247,803 29 October 20 15 (29. 10.20 15) US GM, KE, LR, LS, MW, MZ, NA, RW, SD, SL, ST, SZ, TZ, UG, ZM, ZW), Eurasian (AM, AZ, BY, KG, KZ, RU, (71) Applicant: GLAXOSMITHKLINE CONSUMER TJ, TM), European (AL, AT, BE, BG, CH, CY, CZ, DE, HEALTHCARE HOLDINGS (US) LLC [US/US]; 271 1 DK, EE, ES, FI, FR, GB, GR, HR, HU, IE, IS, IT, LT, LU, Centerville Road, Suite 400, Wilmington, DE 19808 (US). -
( Vaccinium Myrtillus L . ) And
Food Chemistry 354 (2021) 129517 Contents lists available at ScienceDirect Food Chemistry journal homepage: www.elsevier.com/locate/foodchem Analysis of composition, morphology, and biosynthesis of cuticular wax in wild type bilberry (Vaccinium myrtillus L.) and its glossy mutant Priyanka Trivedi a,1, Nga Nguyen a,1, Linards Klavins b, Jorens Kviesis b, Esa Heinonen c, Janne Remes c, Soile Jokipii-Lukkari a, Maris Klavins b, Katja Karppinen d, Laura Jaakola d,e, Hely Haggman¨ a,* a Department of Ecology and Genetics, University of Oulu, FI-90014 Oulu, Finland b Department of Environmental Science, University of Latvia, LV-1004 Riga, Latvia c Centre for Material Analysis, University of Oulu, FI-90014 Oulu, Finland d Department of Arctic and Marine Biology, UiT The Arctic University of Norway, NO-9037 Tromsø, Norway e NIBIO, Norwegian Institute of Bioeconomy Research, NO-1431 Ås, Norway ARTICLE INFO ABSTRACT Keywords: In this study, cuticular wax load, its chemical composition, and biosynthesis, was studied during development of Cuticular wax wild type (WT) bilberry fruit and its natural glossy type (GT) mutant. GT fruit cuticular wax load was comparable Fruit cuticle with WT fruits. In both, the proportion of triterpenoids decreased during fruit development concomitant with Gene expression increasing proportions of total aliphatic compounds. In GT fruit, a higher proportion of triterpenoids in cuticular Glossy type mutant wax was accompanied by a lower proportion of fatty acids and ketones compared to WT fruit as well as lower Triterpenoids Wax composition density of crystalloid structures on berry surfaces. Our results suggest that the glossy phenotype could be caused Chemical compounds studied in this article: by the absence of rod-like structures in GT fruit associated with reduction in proportions of ketones and fatty β-Amyrin (PubChem CID: 73145) acids in the cuticular wax. -
Biochemistry Prologue to Lipids
Paper : 05 Metabolism of Lipids Module: 01 Prologue to Lipids Principal Investigator Dr. Sunil Kumar Khare, Professor, Department of Chemistry, IIT-Delhi Paper Coordinator and Dr. Suaib Luqman, Scientist (CSIR-CIMAP) Content Writer & Assistant Professor (AcSIR) CSIRDr. Vijaya-CIMAP, Khader Lucknow Dr. MC Varadaraj Content Reviewer Prof. Prashant Mishra, Professor, Department of Biochemical Engineering and Biotechnology, IIT-Delhi 1 METABOLISM OF LIPIDS Biochemistry Prologue to Lipids DESCRIPTION OF MODULE Subject Name Biochemistry Paper Name 05 Metabolism of Lipids Module Name/Title 01 Prologue to Lipids 2 METABOLISM OF LIPIDS Biochemistry Prologue to Lipids 1. Objectives To understand what is lipid Why they are important How they occur in nature 2. Concept Map LIPIDS Fatty Acids Glycerol 3. Description 3.1 Prologue to Lipids In 1943, the term lipid was first used by BLOOR, a German biochemist. Lipids are heterogeneous group of compounds present in plants and animal tissues related either actually or potentially to the fatty acids. They are amphipathic molecules, hydrophobic in nature originated utterly or in part by thioesters (carbanion-based condensations of fatty acids and/or polyketides etc) or by isoprene units (carbocation-based condensations of prenols, sterols, etc). Lipids have the universal property of being: i. Quite insoluble in water (polar solvent) ii. Soluble in benzene, chloroform, ether (non-polar solvent) 3 METABOLISM OF LIPIDS Biochemistry Prologue to Lipids Thus, lipids include oils, fats, waxes, steroids, vitamins (A, D, E and K) and related compounds, such as phospholipids, triglycerides, diglycerides, monoglycerides and others, which are allied more by their physical properties than by their chemical assests. -
Aroma Volatile Biosynthesis in Apples Affected by 1-MCP and Methyl Jasmonate
Postharvest Biology and Technology 36 (2005) 61–68 Aroma volatile biosynthesis in apples affected by 1-MCP and methyl jasmonate S. Kondo a, ∗, S. Setha a, D.R. Rudell b, D.A. Buchanan b, J.P. Mattheis b a Graduate School of Applied Biosciences, Hiroshima Prefectural University, Shobara, Hiroshima 727-0023, Japan b Tree Fruit Research Lab, United States Department of Agriculture—ARS, Wenatchee, WA 98801, USA Received 13 August 2004; received in revised form 8 November 2004; accepted 24 November 2004 Abstract Effects of 1-methylcyclopropene (1-MCP), 2-chloroethyl phosphonic acid (ethephon), and methyl jasmonate (MeJA) on production of aroma volatile compounds and ethylene by ‘Delicious’ and ‘Golden Delicious’ apples [Malus sylvestris (L.) Mill. var. domestica (Borkh.) Mansf.] during ripening were investigated. Forty-four volatile compounds in ‘Delicious’ and 40 compounds in ‘Golden Delicious’ were detected. Among volatiles classified as alcohols, esters, ketones, aldehydes, acetic acid, and ␣-farnesene, esters were the most prevalent compounds, followed by alcohols. Aroma volatile production was high in untreated controls and ethephon treatment. Volatile production by 1-MCP-treated fruit was lower compared with untreated controls throughout the evaluation period. The impact of MeJA application on volatile production was cultivar dependent. The combination of ethephon with MeJA reduced volatile production by ‘Delicious’ compared with ethephon only, but this treatment combination stimulated volatile production by ‘Golden Delicious’ with the exception of esters. In general, the effect of MeJA on volatile production was related to the effect of MeJA on internal ethylene concentration. The results suggest that the effect of MeJA on aroma volatiles in apple fruit may be mediated by ethylene. -
Role of Fatty Acid Omega-Hydroxylase 1 and Abscisic Acid in Potato Tuber Suberin Formation
Western University Scholarship@Western Electronic Thesis and Dissertation Repository 5-18-2016 12:00 AM Role of Fatty Acid omega-Hydroxylase 1 and Abscisic Acid in Potato Tuber Suberin Formation Meg Haggitt The University of Western Ontario Supervisor Dr. Mark Bernards The University of Western Ontario Graduate Program in Biology A thesis submitted in partial fulfillment of the equirr ements for the degree in Doctor of Philosophy © Meg Haggitt 2016 Follow this and additional works at: https://ir.lib.uwo.ca/etd Part of the Plant Biology Commons Recommended Citation Haggitt, Meg, "Role of Fatty Acid omega-Hydroxylase 1 and Abscisic Acid in Potato Tuber Suberin Formation" (2016). Electronic Thesis and Dissertation Repository. 4074. https://ir.lib.uwo.ca/etd/4074 This Dissertation/Thesis is brought to you for free and open access by Scholarship@Western. It has been accepted for inclusion in Electronic Thesis and Dissertation Repository by an authorized administrator of Scholarship@Western. For more information, please contact [email protected]. Role of Fatty Acid omega-Hydroxylase 1 (FAωH1) and Abscisic Acid in Potato Tuber Suberin Formation Thesis format: Integrated-Article By Meghan L. Haggitt Department of Biology, Faculty of Science A thesis submitted in partial fulfillment of the requirements for the degree of Doctor of Philosophy SCHOOL OF GRADUATE AND POSTDOCTORAL STUDIES THE UNIVERSITY OF WESTERN ONTARIO LONDON, ONTARIO, CANADA © Meghan L. Haggitt 2016 Abstract Suberin is a complex biopolymer composed of two distinct but covalently-linked domains. The first domain is composed of polymerized phenolic monomers, whereas the second domain is predominately fatty acid derivatives esterified with glycerol. -
WO 2016/105530 Al 30 June 2016 (30.06.2016) W P O P C T
(12) INTERNATIONAL APPLICATION PUBLISHED UNDER THE PATENT COOPERATION TREATY (PCT) (19) World Intellectual Property Organization International Bureau (10) International Publication Number (43) International Publication Date WO 2016/105530 Al 30 June 2016 (30.06.2016) W P O P C T (51) International Patent Classification: AO, AT, AU, AZ, BA, BB, BG, BH, BN, BR, BW, BY, A61K 47/10 (2006.01) A61K 47/22 (2006.01) BZ, CA, CH, CL, CN, CO, CR, CU, CZ, DE, DK, DM, A61K 47/12 (2006.01) DO, DZ, EC, EE, EG, ES, FI, GB, GD, GE, GH, GM, GT, HN, HR, HU, ID, IL, IN, IR, IS, JP, KE, KG, KN, KP, KR, (21) International Application Number: KZ, LA, LC, LK, LR, LS, LU, LY, MA, MD, ME, MG, PCT/US20 15/000302 MK, MN, MW, MX, MY, MZ, NA, NG, NI, NO, NZ, OM, (22) International Filing Date: PA, PE, PG, PH, PL, PT, QA, RO, RS, RU, RW, SA, SC, 23 December 2015 (23. 12.2015) SD, SE, SG, SK, SL, SM, ST, SV, SY, TH, TJ, TM, TN, TR, TT, TZ, UA, UG, US, UZ, VC, VN, ZA, ZM, ZW. (25) Filing Language: English (84) Designated States (unless otherwise indicated, for every (26) Publication Language: English kind of regional protection available): ARIPO (BW, GH, (30) Priority Data: GM, KE, LR, LS, MW, MZ, NA, RW, SD, SL, ST, SZ, 62/096,148 23 December 2014 (23. 12.2014) US TZ, UG, ZM, ZW), Eurasian (AM, AZ, BY, KG, KZ, RU, TJ, TM), European (AL, AT, BE, BG, CH, CY, CZ, DE, (72) Inventor; and DK, EE, ES, FI, FR, GB, GR, HR, HU, IE, IS, IT, LT, LU, (71) Applicant : HOFFMAN, Steven [US/US]; 15 Knichel LV, MC, MK, MT, NL, NO, PL, PT, RO, RS, SE, SI, SK, Road, Mahwah, NJ 07430 (US). -
Molecular Tracers and Untargeted Characterization of Water Soluble Organic Compounds in Polar Ice for Climate Change Studies
PhD Thesis in SCIENCE AND MANAGEMENT OF CLIMATE CHANGE Molecular tracers and untargeted characterization of water soluble organic compounds in polar ice for climate change studies. PhD Candidate Ornela Karroca Supervisor Prof. Andrea Gambaro Co-Supervisor Dr. Roberta Zangrando 1 GLOSSARY 1. ABSTRACT ........................................................................................................................................ 4 2. INTRODUCTION ............................................................................................................................... 5 3. THESIS GOALS ................................................................................................................................ 15 4. EXPERIMENTAL SECTION ............................................................................................................... 16 4.1 Reagents and standard solutions ............................................................................................ 16 4.2 Quantitative analyses .............................................................................................................. 17 4.2.1 Instrumentation and working conditions .......................................................................... 17 4.2.2 Amino acids and phenolic compounds: Method validation .............................................. 21 4.2.2.1 Chromatographic separation ..................................................................................... 21 4.2.2.2 Quality control........................................................................................................... -
Obese Mice with Dyslipidemia Exhibit Meibomian Gland Hypertrophy and Alterations in Meibum Composition and Aqueous Tear Production
International Journal of Molecular Sciences Article Obese Mice with Dyslipidemia Exhibit Meibomian Gland Hypertrophy and Alterations in Meibum Composition and Aqueous Tear Production Eugene A. Osae 1,* , Tiffany Bullock 2, Madhavi Chintapalati 2, Susanne Brodesser 3 , Samuel Hanlon 1, Rachel Redfern 1, Philipp Steven 4 , C. Wayne Smith 2, Rolando E. Rumbaut 2,5 and Alan R. Burns 1 1 College of Optometry, University of Houston, Houston, TX 77204, USA; [email protected] (S.H.); [email protected] (R.R.); [email protected] (A.R.B.) 2 Children’s Nutrition Research Center, Baylor College of Medicine, Houston, TX 77030, USA; [email protected] (T.B.); [email protected] (M.C.); [email protected] (C.W.S.); [email protected] (R.E.R.) 3 CECAD Research Center, Lipidomics/Metabolomics Facility, University of Cologne, 50931 Cologne, Germany; [email protected] 4 Department of Ophthalmology, Division for Dry-Eye and Ocular GvHD, Medical Faculty, University of Cologne, 50937 Cologne, Germany; [email protected] 5 Center for Translational Research on Inflammatory Diseases (CTRID), Michael E. DeBakey Veterans Affairs Medical Center, Houston, TX 77030, USA * Correspondence: [email protected]; Tel.: +1-346-317-6273 Received: 14 October 2020; Accepted: 16 November 2020; Published: 20 November 2020 Abstract: Background: Dyslipidemia may be linked to meibomian gland dysfunction (MGD) and altered meibum lipid composition. The purpose was to determine if plasma and meibum cholesteryl esters (CE), triglycerides (TG), ceramides (Cer) and sphingomyelins (SM) change in a mouse model of diet-induced obesity where mice develop dyslipidemia. Methods: Male C57/BL6 mice (8/group, age = 6 wks) were fed a normal (ND; 15% kcal fat) or an obesogenic high-fat diet (HFD; 42% kcal fat) for 10 wks. -
Alpha Omega Cohort Data Dictionary
Alpha Omega Cohort Data dictionary - Food Frequency Questionnaire - Eveline Waterham Alpha Omega Cohort – Data Dictionary Table of contents 1 Dietary pattern ...................................................................................................................................................... 3 2 Bread and wheat products ................................................................................................................................... 3 3 Butter and margarine ........................................................................................................................................... 4 4 Bread toppings ..................................................................................................................................................... 4 5 Breakfast and milk products ................................................................................................................................. 5 6 Coffee and tea ...................................................................................................................................................... 5 7 Diner ..................................................................................................................................................................... 6 7.1 Starch base ....................................................................................................................................... 6 7.2 Soups ............................................................................................................................................... -
Open Thesis.Pdf
THE PENNSYLVANIA STATE UNIVERSITY SCHREYER HONORS COLLEGE DEPARTMENT OF VETERINARY AND BIOMEDICAL SCIENCE EFFECTS OF DIETARY LINOLEIC ACID ON CONVERSION OF OMEGA-3 FATTY ACIDS TO LONG CHAIN FATTY ACIDS ELIZABETH ANN FLAHERTY FALL 2018 A thesis submitted in partial fulfillment of the requirements for a baccalaureate degree in Veterinary and Biomedical Science with honors in Veterinary and Biomedical Science Reviewed and approved* by the following: Dr. Kevin Harvatine Associate Professor of Nutritional Physiology Thesis Supervisor Dr. Robert VanSaun Professor of Veterinary Science Honors Adviser * Signatures are on file in the Schreyer Honors College. i ABSTRACT With the modern diet that is high in total fats, high in omega-6 fatty acids (FA), and low in omega-3 FA, there is a high prevalence of metabolic and cardiovascular disease. Certain dietary acids are beneficial, while others may contribute to these disease processes. Eggs are an important part of the human diet as they are protein and nutrient dense and are a good source of vitamins and minerals. It is possible to manipulate the nutrients and composition of FA in the eggs by modifying the balance of the hens diets. This makes them a good target for experiments with fatty acids (FA). A diet high in omega-3 (n-3) FA has many beneficial effects including plasma lipid reduction, reduction in some types of cancer mortality, anti-inflammatory effects, antiarrhythmic effects, antithrombotic effects, antiatheromatous effects, and less severe manifestations of autoimmune diseases and inflammatory diseases. Due to the benefits of n-3 FA, it is important to understand the effects of oleic acid and linoleic acid (LA; C18:2n-6) on the deposition of n-3 FA in egg yolks. -
Waradur® Wax Additives Improve Your Plastic
| plastic series WARADUR® WAX ADDITIVES Higher tensile Proper Flow Reduced strength / dispersion improvement ejection force impact strength IMPROVE YOUR PLASTIC PRODUCTION WARADUR® S | WARAMONT CA | WARADUR® GE WARADUR® GSM | WARADUR® E | WARADUR® ESM WARADUR® OP | WARADUR® OPplus | WARADUR® GSA OVERVIEW With almost 120 years of experience in the production of VOELPKER Plastic Series: At the heart of the WARADUR® (the montan and specialty waxes, VOELPKER is one of the most subject of this brochure) and CEVO® special wax additives from experienced wax producers in Europe. VOELPKER develops and VOELPKER is their unique multifunctionality. This enables the produces tailor-made wax derivatives whose chemical functio- simultaneous resolution of different processing problems. It nalities and application properties are precisely adapted to the leads to improved surface quality and filler or pigment dispersi- specific use. on, improved flow properties and the reduction of friction peaks. WARADUR® montan wax derivatives also enable the production of particularly high-quality compounds containing recyclate. WARADUR® WAX ADDITIVES - PERFORMANCE AND MULTIFUNCTIONALITY INTRODUCTION WARADUR® montan waxes are used as high performance addi- challenging tasks in the engineering plastics field where high tives in the plastics industry, due to their unique properties and demands are placed on functionality, optical appearance and versatility. Montan wax derivatives serve for example as com- cycle time reduction. WARADUR® montan waxes in addition bined external