Origin of Dipping Structures in Fast-Spreading Oceanic Lower Crust Offshore Alaska Imaged by Multichannel Seismic Data ∗ Anne Bécel A, , Donna J
Total Page:16
File Type:pdf, Size:1020Kb
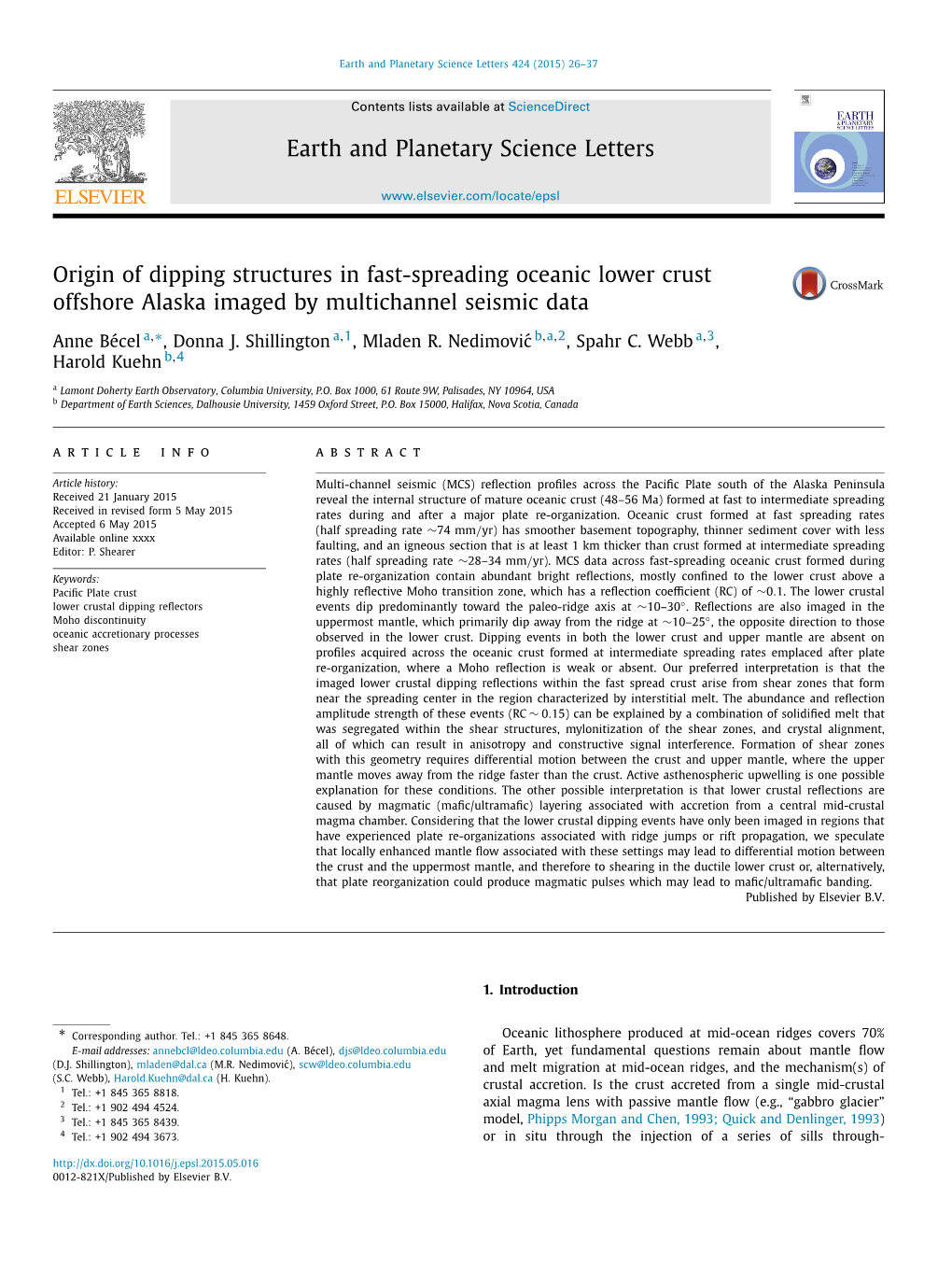
Load more
Recommended publications
-
Paleomagnetic Reconstruction in the Troodos Ophiolite Gabbro
The oceanic crust in 3D: Paleomagnetic reconstruction in the Troodos ophiolite gabbro Abstract The Troodos complex, Cyprus, provides an opportunity to study the structural configuration along a fossil intersection of a spreading axis and a transform fault. We complement studies at Troodos that have reconstructed the brittle deformation of the upper crust by new paleomagnetic data from the gabbro suite. The gabbro suite is exposed at the extinct spreading axis continuing the Solea graben toward the intersection with the fossil Arakapas oceanic transform. This is a unique exposure of deep crustal rocks formed at both an inside-corner and an outside-corner of a ridge- transform intersection. Remanence directions from gabbros (24 sites) were used as indicators for rigid body rotation. The spatial distribution of rotation axes allow recognition of three regions to which deformation is partitioned: 1) a western region (outside corner) that experienced primarily tilt about horizontal axis 2) a central region with minor rotation and, 3) an eastern area (inside corner) where vertical axis rotations are dominant. The absence of significant rotation in the 6 km-wide central domain together with its location between the inside- and the outside corner uncover the root of a fossil axial volcanic zone, a zone sufficiently hot so the upper crust can decouple from the substrate. Clockwise rotation in the gabbro increases from the axial zone eastward, similar to that in the overlying dikes, indicating coupling of the lower crust with the brittle upper oceanic crust. The transition from the decoupled layers of sheeted dikes and gabbro in the axial-zone to the dikes- gabbro coupling in the inside corner is in keeping with deepening of the brittle-ductile transition from the dike-gabbro boundary into the lower crust away from the axial zone. -
Mantle Hydration and Cl-Rich Fluids in the Subduction Forearc Bruno Reynard1,2
Reynard Progress in Earth and Planetary Science (2016) 3:9 Progress in Earth and DOI 10.1186/s40645-016-0090-9 Planetary Science REVIEW Open Access Mantle hydration and Cl-rich fluids in the subduction forearc Bruno Reynard1,2 Abstract In the forearc region, aqueous fluids are released from the subducting slab at a rate depending on its thermal state. Escaping fluids tend to rise vertically unless they meet permeability barriers such as the deformed plate interface or the Moho of the overriding plate. Channeling of fluids along the plate interface and Moho may result in fluid overpressure in the oceanic crust, precipitation of quartz from fluids, and low Poisson ratio areas associated with tremors. Above the subducting plate, the forearc mantle wedge is the place of intense reactions between dehydration fluids from the subducting slab and ultramafic rocks leading to extensive serpentinization. The plate interface is mechanically decoupled, most likely in relation to serpentinization, thereby isolating the forearc mantle wedge from convection as a cold, potentially serpentinized and buoyant, body. Geophysical studies are unique probes to the interactions between fluids and rocks in the forearc mantle, and experimental constrains on rock properties allow inferring fluid migration and fluid-rock reactions from geophysical data. Seismic velocities reveal a high degree of serpentinization of the forearc mantle in hot subduction zones, and little serpentinization in the coldest subduction zones because the warmer the subduction zone, the higher the amount of water released by dehydration of hydrothermally altered oceanic lithosphere. Interpretation of seismic data from petrophysical constrain is limited by complex effects due to anisotropy that needs to be assessed both in the analysis and interpretation of seismic data. -
Physiography of the Seafloor Hypsometric Curve for Earth’S Solid Surface
OCN 201 Physiography of the Seafloor Hypsometric Curve for Earth’s solid surface Note histogram Hypsometric curve of Earth shows two modes. Hypsometric curve of Venus shows only one! Why? Ocean Depth vs. Height of the Land Why do we have dry land? • Solid surface of Earth is Hypsometric curve dominated by two levels: – Land with a mean elevation of +840 m = 0.5 mi. (29% of Earth surface area). – Ocean floor with mean depth of -3800 m = 2.4 mi. (71% of Earth surface area). If Earth were smooth, depth of oceans would be 2450 m = 1.5 mi. over the entire globe! Origin of Continents and Oceans • Crust is formed by differentiation from mantle. • A small fraction of mantle melts. • Melt has a different composition from mantle. • Melt rises to form crust, of two types: 1) Oceanic 2) Continental Two Types of Crust on Earth • Oceanic Crust – About 6 km thick – Density is 2.9 g/cm3 – Bulk composition: basalt (Hawaiian islands are made of basalt.) • Continental Crust – About 35 km thick – Density is 2.7 g/cm3 – Bulk composition: andesite Concept of Isostasy: I If I drop a several blocks of wood into a bucket of water, which block will float higher? A. A thick block made of dense wood (koa or oak) B. A thin block made of light wood (balsa or pine) C. A thick block made of light wood (balsa or pine) D. A thin block made of dense wood (koa or oak) Concept of Isostasy: II • Derived from Greek: – Iso equal – Stasia standing • Density and thickness of a body determine how high it will float (and how deep it will sink). -
The Nature of the Mohorovicic Discontinuity, a Compromise 1
JOURNAL OF GEOPHYSICAL RESEARCH VOL. 68, No. 15 AUGUST 1, 1963 The Nature of the Mohorovicic Discontinuity, A Compromise 1 PETER J. WYLLIE Department of Geochemistry and Minemlogy Pennsylvania State Universit.y, University Park Abstract available . The experimental data and steady-state calculations make it difficult M to explain the discontinuity beneath both oceans and continents on the basis of the same change. The phase oceanic M discontinuity may be a chemical discontinuity between basalt and peridotite , and a similar chemical discontinuity ma,y thus be expected b�neath the conti nents. Since ayailable experimental data place the basalt-eclogite phase change at about thE' same depth as the continental M discontinuity, intersections may exist between a zone of chemical discontinuity and a phase transition zone, the transition being either basalt-ecloO"ite or feldspathic peridotite-garnet peridotite. Detection of the latter transition by seismic t�'h niques ma,y be difficult. The M discontinuity could therefore represent the basalt-eclogite phase change in some localities (e.g. mountain belts) and the chemical discontinuity in others (e.g. oceans and continental shields). Variations in the depth to the chemical discontinuity and in the positions of geoisotherms produce great flexibility in oro genetic models. Interse; tions between the two zones at depth could be reflected at the surface by major fault zones separating large structural blocks of different elevations. Chemical discontinuity. The conyentional termediate between dunite and basalt is view that the Moho discontinuity at the base of qualitatively reasonable for the mantle. the crust is caused by a chemical change from Ringwood [1962a, c] adopted a specific model basaltic rock to peridotite has been expounded compounded from the concepts advanced and recently by Hess [1955], Wager [1958], and developed by many petrologists and geochem Harris and Rowell [1960]. -
Density of Oceanic Crust
Density of Oceanic Crust Introduction Procedures Certain properties of a substance are both distinctive and Part 1 relatively easy to determine. Density, the ratio between 1. Complete Report Sheet 1 by calculating the missing a sample’s mass and volume at a specific temperature densities. How will you deal with the differences in and pressure (like standard ambient temperature and significant digits? Make sure to include units in your pressure), is one such property. Regardless of the size of a answers. sample, the density of a substance will always remain the 2. Using the depths and densities from your chart, plot same. The density of a rock sample can, therefore, be used a graph on your own paper (or use an electronic in the identification process. graphing tool) and title it Depth vs. Density. Hint: Think about independent and dependent variables. Using a While density may vary only slightly from rock to rock, blue colored pencil, draw a line of best fit from the XY- detailed sampling and correlation with other factors like intercept through the plotted points. depth may reveal important information about the history of a core, or may help to improve the use of seismic Part 2 profiles. The average density of oceanic crust is 3.0 g/cm3, 1. Find the mass and volume for each of the four continental while continental crust has an average of 2.7 g/cm3. samples as instructed by your teacher. How will you deal with error in the laboratory? How about significant Objectives digits? Record your answers in the space provided. -
Peter Castro | Michael E. Huber
TEACHER’S MANUAL Marine Science Peter Castro | Michael E. Huber SECOND EDITION Waves and Tides Chapter Introduce the BIG IDEA Waves and Tides Have students watch a short video clip of ocean waves 4 breaking on the shore. Have them make observations about the movement of water as the wave breaks and then retreats back to the ocean. If any students have been to the ocean, have them share their experience of the water and the waves. Pose students these questions and let them discuss responses in small groups. 1. Why does the size of a wave change as it approaches the shore? 2. What factors affect the tides and make the tide come in and leave again? 3. What hardships do marine organisms deal with that live in the intertidal zones? Small groups can share back with the whole group on key GO ONLINE To access points their groups discussed. inquiry and investigative activities to accompany this chapter. Ocean Section Pacing Main Idea and Key Questions Literacy Labs and Activities Standards 4.1 Introduc- 1 day Waves carry energy across the sea surface but Key Questions Activity: tion to Wave do not transport water. Making Waves Energy and 1. What are the three most common generating Motion forces of waves? 2. What are the two restoring forces that cause the water surface to return to its undisturbed state? 4.2 Types of 2 days There are several types of waves, each with its 2.d, 5.j, 6.f, Laboratory Manual: Making Waves own characteristics and different ways of 7.e Waves (p. -
Ophiolites and Oceanic Crust: New Insights from Field Studies and the Ocean Drilling Program
Ophiolites and Oceanic Crust: New Insights from Field Studies and the Ocean Drilling Program Geological Society of America Special Paper 349 Edited by Yildirim Dilek, Eldridge Moores, Don Elthon, and Adolphe Nicolas "Ophiolites are geological windows into the history of the Earth and Earth processes," Dilek and Moores said. "They provide important clues to how ocean basins formed and disappeared in the past and how the dynamic planet Earth's paleogeography (distribution of continental masses and oceans) looked many millions of years ago. The discovery of copper in ophiolitic volcanic rocks in the Mediterranean region ushered in the Bronze Age in the history of human civilizations. Studies of ophiolites have advanced the methods and theories of geology for more than 200 years. This book presents state-of-the-art information on the significance of ophiolites in studying different aspects of the Earth's history." Since 1972, when the first GSA Penrose Conference on Ophiolites was convened, these unique features have galvanized multinational and multidisciplinary efforts to study and decipher these complexes and their significance for understanding oceanic lithosphere formation. The second Penrose Conference on ophiolites in 1998 brought together 86 experts in structural geology, tectonics, geophysics, petrology, and geochemistry to explore new advances and discoveries concerning ophiolites and related drilling from the oceanic crust. Special Paper 349 includes 39 papers from this conference plus updated information on the evolution of ophiolites and modern oceanic crust, and integrates new interdisciplinary data from ocean drilling and other studies of in situ oceanic lithosphere with new results from field studies of ophiolite complexes around the world. -
Global Sea Level Rise Scenarios for the United States National Climate Assessment
Global Sea Level Rise Scenarios for the United States National Climate Assessment December 6, 2012 PhotoPhPhototo prpprovidedrovovidideedd bbyy thtthehe GrGGreaterreaeateter LaLLafourcheafofoururchche PoPortrt CoComommissionmimissssioion NOAA Technical Report OAR CPO-1 GLOBAL SEA LEVEL RISE SCENARIOS FOR THE UNITED STATES NATIONAL CLIMATE ASSESSMENT Climate Program Office (CPO) Silver Spring, MD Climate Program Office Silver Spring, MD December 2012 UNITED STATES NATIONAL OCEANIC AND Office of Oceanic and DEPARTMENT OF COMMERCE ATMOSPHERIC ADMINISTRATION Atmospheric Research Dr. Rebecca Blank Dr. Jane Lubchenco Dr. Robert Dietrick Acting Secretary Undersecretary for Oceans and Assistant Administrator Atmospheres NOTICE from NOAA Mention of a commercial company or product does not constitute an endorsement by NOAA/OAR. Use of information from this publication concerning proprietary products or the tests of such products for publicity or advertising purposes is not authorized. Any opinions, findings, and conclusions or recommendations expressed in this material are those of the authors and do not necessarily reflect the views of the National Oceanic and Atmospheric Administration. Report Team Authors Adam Parris, Lead, National Oceanic and Atmospheric Administration Peter Bromirski, Scripps Institution of Oceanography Virginia Burkett, United States Geological Survey Dan Cayan, Scripps Institution of Oceanography and United States Geological Survey Mary Culver, National Oceanic and Atmospheric Administration John Hall, Department of Defense -
Drilling the Crust at Mid-Ocean Ridges
or collective redistirbution of any portion of this article by photocopy machine, reposting, or other means is permitted only with the approval of Th e Oceanography Society. Send all correspondence to: [email protected] or Th eOceanography PO Box 1931, Rockville, USA.Society, MD 20849-1931, or e to: [email protected] Oceanography correspondence all Society. Send of Th approval portionthe ofwith any articlepermitted only photocopy by is of machine, reposting, this means or collective or other redistirbution articleis has been published in Th SPECIAL ISSUE FEATURE Oceanography Drilling the Crust Threproduction, Republication, systemmatic research. for this and teaching article copy to use in reserved. e is rights granted All OceanographyPermission Society. by 2007 eCopyright Oceanography Society. journal of Th 20, Number 1, a quarterly , Volume at Mid-Ocean Ridges An “in Depth” perspective BY BENOÎT ILDEFONSE, PETER A. RONA, AND DONNA BLACKMAN In April 1961, 13.5 m of basalts were drilled off Guadalupe In the early 1970s, almost 15 years after the fi rst Mohole Island about 240 km west of Mexico’s Baja California, to- attempt, attendees of a Penrose fi eld conference (Confer- gether with a few hundred meters of Miocene sediments, in ence Participants, 1972) formulated the concept of a layered about 3500 m of water. This fi rst-time exploit, reported by oceanic crust composed of lavas, underlain by sheeted dikes, John Steinbeck for Life magazine, aimed to be the test phase then gabbros (corresponding to the seismic layers 2A, 2B, for the considerably more ambitious Mohole project, whose and 3, respectively), which themselves overlay mantle perido- objective was to drill through the oceanic crust down to tites. -
Cretaceous History of Pacific Basin Guyot Reefs: a Reappraisal Based on Geothermal Endo-Upwelling
PALAEOZ1c01061 ELSEVIER Palaeogeography, Palaeoclimatology, Palaeoecology 112 (1994) 239-260 Cretaceous history of Pacific Basin guyot reefs: a reappraisal based on geothermal endo-upwelling Francis Rougerie a, J.A. Fagerstrom a QRSTQM, B. P. 529, Papeete, Tahiti, French Polynesia Departinent of GeologicalSciences, The Uiziversity of Colorado, Boulder, CO 80309, USA Received 23 July, 1992; revised and accepted 15 April 1994 Abstract The mid-Cretaceous histories (origin, growth, death) of algal-rudist-stromatoporoid reef communities located on many Pacific Basin guyots are complex and controversial. These shallow water, tropical communities originated on volcanic edifices extruded during the Barremian-Albian, grew upward during edifice subsidenceftransgression throughout the Aptian, Albian and Cenomanian and several of them died almost synchronously near the Cenomanian- Turonian boundary. During their periods of origin and growth, we postulate that the reef ecosystems received dissolved oxygen by wave surge and nutrients by geothermal endo-upwelling. By this process oceanic waters of intermediate depth (approx. 500-1500 m) were: (a) drawn into the weathered and fractured volcanic summit and lower part of the older reef and driven upward through the porous framework by the remnant geothermal gradient of the volcanic foundation and (b) emerged at the reef surface to support the high metabolism of the living community. The death of most of the reefs near the Cenomanian-Turonian boundary approximately coincides with the most intense oceanic anoxic event (Om)in Pacific Ocean history. During this OAE the chemistry of the endo-upwelled fluids arriving at the reef surface changed from nutrient/oxygen-rich to dysoxic-anoxic-toxic, and killed the community. -
Subduction Zones
SUBDUCTION ZONES Robert J. Stern Geosciences Department University of Texas at Dallas Richardson, Texas, USA Received 24 January 2001; revised 24 June 2002; accepted 11 November 2002; published 31 December 2002. [1] Subduction zones are where sediments, oceanic continental crust, and earthquakes caused by the down- crust, and mantle lithosphere return to and reequilibrate going plate present a growing hazard to society. This with Earth’s mantle. Subduction zones are interior ex- overview summarizes our present understanding of sub- pressions of Earth’s 55,000 km of convergent plate mar- duction zones, using perspectives of the incoming plate, gins and are the geodynamic system that builds island downgoing plate, mantle wedge, and arc-trench com- arcs. Excess density of the mantle lithosphere in subduc- plex. Understanding the operation of subduction zones tion zones provides most of the power needed to move stands as one of the great challenges facing the Earth the plates while inducing convection in the overriding sciences in the 21st century and will require the efforts of mantle wedge. Asthenospheric mantle sucked toward global interdisciplinary teams. INDEX TERMS: 1010 Geochemis- the trench by the sinking slab interacts with water and try: Chemical evolution; 1030 Geochemistry: Geochemical cycles incompatible elements rising from the sinking plate, and (0330); 7299 Seismology: General or miscellaneous; 8120 Tectono- this interaction causes the mantle to melt. These melts physics: Dynamics of lithosphere and mantle—general; 8155 Tectono- rise vertically through downwelling mantle to erupt at physics: Plate motions—general; KEYWORDS: island arc, Wadati-Be- arc volcanoes. Subduction zones are thus interior Earth nioff Zone, subduction, tectonics systems of unparalleled scale and complexity. -
Deep-Ocean Basins
Chapter23 The Ocean Basins ChapterCh t Outline Outli ne 1 ● The Water Planet Divisions of the Global Ocean Exploration of the Ocean 2 ● Features of the Ocean Floor Continental Margins Deep-Ocean Basins 3 ● Ocean-Floor Sediments Sources of Deep Ocean– Basin Sediments Physical Classification of Sediments Why It Matters Oceans cover more than 70 percent of Earth’s surface. Oceans interact with the atmosphere to influence weather and climate. Exploring and analyzing data about the chemistry of ocean water, the geology of the ocean floor, marine ecosystems, and the physics of water movement is critical to understanding natural processes on Earth. 634 Chapter 23 hhq10sena_obacho.inddq10sena_obacho.indd 663434 PDF 88/1/08/1/08 11:09:46:09:46 PPMM Inquiry Lab 20 min Sink or Float? Fill the bottom half of 3-L soda bottle with water to within about 3 inches from the top and place it on a plastic or metal tray to catch any spills. Using small (3-oz) plastic cups, with some paper clips and metal nuts for ballast, see if you can get a cup to sink with air still trapped inside, simulating a submersible. You may need something pointed to make holes in the cups. Questions to Get You Started 1. What is buoyancy and why is it so important for maritime travel? 2. Compare techniques for sinking the cup. Does one method consistently work better than others? 3. What challenges do engineers face when designing submersibles? 635 hhq10sena_obacho.inddq10sena_obacho.indd 663535 PDF 88/1/08/1/08 11:09:58:09:58 PPMM These reading tools will help you learn the material in this chapter.