Are Ultraluminous X-Ray Sources Intermediate Mass
Total Page:16
File Type:pdf, Size:1020Kb
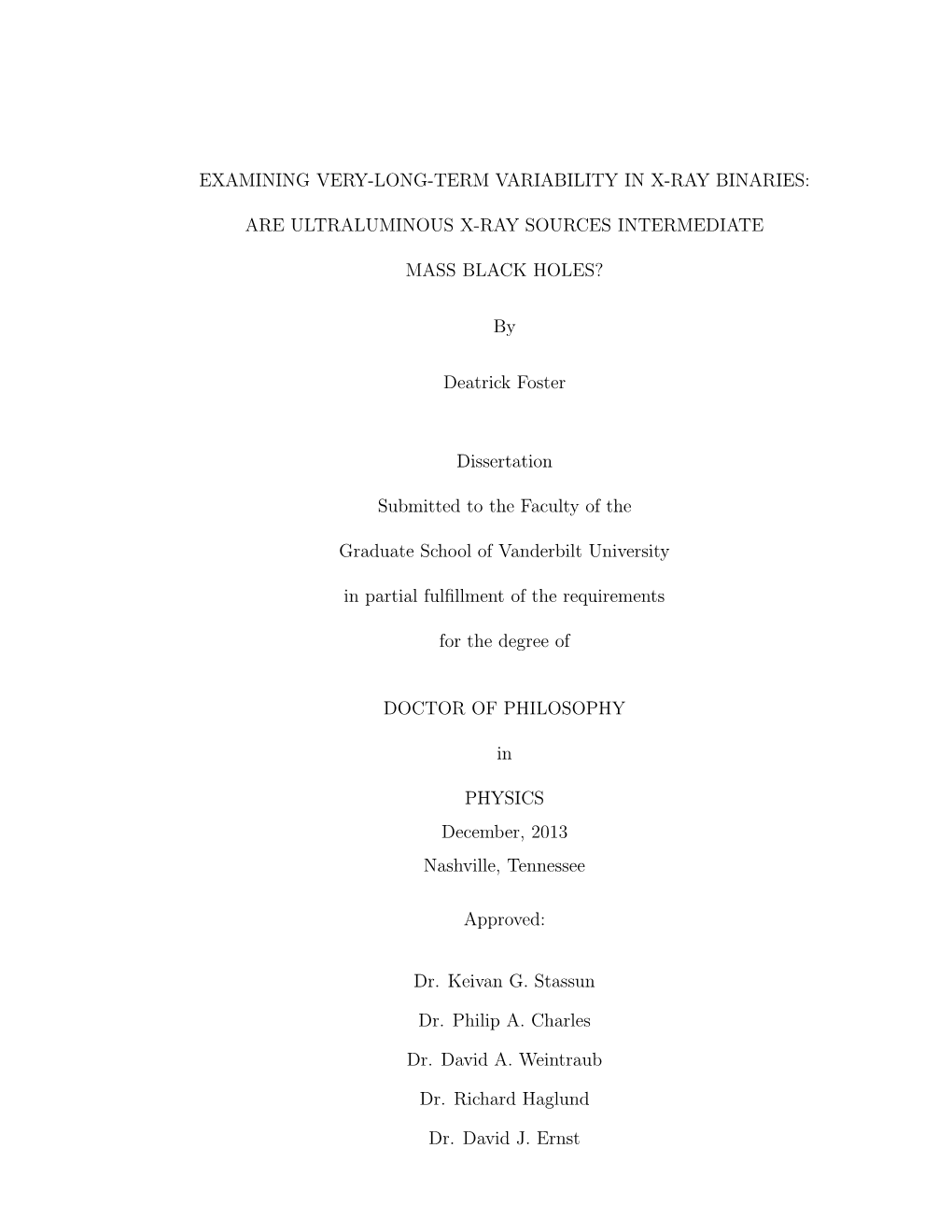
Load more
Recommended publications
-
A Fuse Survey of the Rotation Rates of Very Massive Stars in the Small and Large Magellanic Clouds
The Astrophysical Journal, 700:844–858, 2009 July 20 doi:10.1088/0004-637X/700/1/844 C 2009. The American Astronomical Society. All rights reserved. Printed in the U.S.A. A FUSE SURVEY OF THE ROTATION RATES OF VERY MASSIVE STARS IN THE SMALL AND LARGE MAGELLANIC CLOUDS Laura R. Penny1 and Douglas R. Gies2 1 Department of Physics and Astronomy, The College of Charleston, Charleston, SC 29424, USA; [email protected] 2 Center for High Angular Resolution Astronomy and Department of Physics and Astronomy, Georgia State University, P.O. Box 4106, Atlanta, GA 30302-4106, USA; [email protected] Received 2009 February 3; accepted 2009 May 26; published 2009 July 6 ABSTRACT We present projected rotational velocity values for 97 Galactic, 55 SMC, and 106 LMC O-B type stars from archival FUSE observations. The evolved and unevolved samples from each environment are compared through the Kolmogorov–Smirnov test to determine if the distribution of equatorial rotational velocities is metallicity dependent for these massive objects. Stellar interior models predict that massive stars with SMC metallicity will have significantly reduced angular momentum loss on the main sequence compared to their Galactic counterparts. Our results find some support for this prediction but also show that even at Galactic metallicity, evolved and unevolved massive stars have fairly similar fractions of stars with large V sin i values. Macroturbulent broadening that is present in the spectral features of Galactic evolved massive stars is lower in the LMC and SMC samples. This suggests the processes that lead to macroturbulence are dependent upon metallicity. -
The X-Ray Universe 2011 @ Berlin, 27-30 June 2011
The X-ray Universe 2011 @ Berlin, 27-30 June 2011 List of poster presentations: Topic A: Stars, star-forming regions, planetary and cometary studies Poster Title Author A01 Young stars around the Horsehead nebula Albacete Colombo A02 A very deep X-ray look into the young stellar cluster IC348 Alexander A03 X-ray emission from protostellar jet HH 154: first evidence of a diamond shock? Bonito A04 The nature and importance of the large spread in T Tauri X-ray luminosities Drake A05 The young open cluster around 25 Ori Franciosini A06 Chandra/ACIS-I study of the young stellar population of the Eagle Nebula Guarcello A07 Cluster members and disk fraction of CygnusOB2 Guarcello A08 Constraining the ages of X-ray sources in NGC 922 Jackson A09 An XMM-Newton vision of the NGC 2023 cluster and its surroundings Lopez-Garcia A10 Optical follow-up of the stellar content of the XBSS Lopez-Santiago A11 Quantifying the relation between UV and X-ray flux in nearby M stars Marino A12 X-ray emission from brown dwarf candidates in Cha I Martinez-Arnaiz A13 Star-planet interactions in X-rays - mimicked by selection effects? Poppenhaeger A14 The LETG spectrum of delta Ori Raassen A15 A look at the high Galactic latitude O-type star HD93521 with XMM-Newton Rauw A16 X-ray emission from protostellar jets Schneider A17 Giant HII regions in M 101: Hα-line spectra and X-ray property Sun A18 The decay of stellar dynamo activity and the rotation-activity relation Wright Topic B: Interacting binary systems, (Galactic) black holes & micro-quasars Poster Title Author -
Journal Reprints-Astronomy/Download/8526
SPACE PATTERNS AND PAINTINGS (published in the "Engineer" magazine No. 8-9, 2012) Sagredo. - If the end of the pen, which was on the ship during my entire voyage from Venice to Alexandretta, were able to leave a visible trace of its entire path, then what kind of trace, what mark, what line would it leave? Simplicio. - I would leave a line stretching from Venice to the final place, not completely straight, or rather, extended in the form of an arc of a circle, but more or less wavy, depending on how much the ship swayed along the way ... Sagredo. - If, therefore, the artist, upon leaving the harbor, began to draw with this pen on a sheet of paper and continued drawing until Alexandretta, he could get from his movement a whole picture of figures ... at least a trace left ... by the end of the pen would be nothing more than a very long and simple line. G. Galileo "Dialogue on the two main systems of the world" Space has been giving astronomers surprise after surprise lately. A number of mysterious space objects were discovered that were not predicted by astrophysics and contradicted it. The more advanced observation methods become, the more such surprises. At one time, I. Shklovsky argued that the discovery of such "cosmic wonders" would confirm the reality of extraterrestrial intelligence, its enormous technical capabilities. But in reality, the "cosmic miracles" showed the backwardness of terrestrial science, which the opium of relativism smiled so much that now it cannot clearly explain ordinary cosmic phenomena caused by natural causes. -
Thunderkat: the Meerkat Large Survey Project for Image-Plane Radio Transients
ThunderKAT: The MeerKAT Large Survey Project for Image-Plane Radio Transients Rob Fender1;2∗, Patrick Woudt2∗ E-mail: [email protected], [email protected] Richard Armstrong1;2, Paul Groot3, Vanessa McBride2;4, James Miller-Jones5, Kunal Mooley1, Ben Stappers6, Ralph Wijers7 Michael Bietenholz8;9, Sarah Blyth2, Markus Bottcher10, David Buckley4;11, Phil Charles1;12, Laura Chomiuk13, Deanne Coppejans14, Stéphane Corbel15;16, Mickael Coriat17, Frederic Daigne18, Erwin de Blok2, Heino Falcke3, Julien Girard15, Ian Heywood19, Assaf Horesh20, Jasper Horrell21, Peter Jonker3;22, Tana Joseph4, Atish Kamble23, Christian Knigge12, Elmar Körding3, Marissa Kotze4, Chryssa Kouveliotou24, Christine Lynch25, Tom Maccarone26, Pieter Meintjes27, Simone Migliari28, Tara Murphy25, Takahiro Nagayama29, Gijs Nelemans3, George Nicholson8, Tim O’Brien6, Alida Oodendaal27, Nadeem Oozeer21, Julian Osborne30, Miguel Perez-Torres31, Simon Ratcliffe21, Valerio Ribeiro32, Evert Rol6, Anthony Rushton1, Anna Scaife6, Matthew Schurch2, Greg Sivakoff33, Tim Staley1, Danny Steeghs34, Ian Stewart35, John Swinbank36, Kurt van der Heyden2, Alexander van der Horst24, Brian van Soelen27, Susanna Vergani37, Brian Warner2, Klaas Wiersema30 1 Astrophysics, Department of Physics, University of Oxford, UK 2 Department of Astronomy, University of Cape Town, South Africa 3 Department of Astrophysics, Radboud University, Nijmegen, the Netherlands 4 South African Astronomical Observatory, Cape Town, South Africa 5 ICRAR, Curtin University, Perth, Australia 6 University -
Powerful Jets from Radiatively Efficient Disks, a Decades-Old Unresolved Problem in High Energy Astrophysics
galaxies Article Powerful Jets from Radiatively Efficient Disks, a Decades-Old Unresolved Problem in High Energy Astrophysics Chandra B. Singh 1,*,†, David Garofalo 2,† and Benjamin Lang 3 1 South-Western Institute for Astronomy Research, Yunnan University, University Town, Chenggong, Kunming 650500, China 2 Department of Physics, Kennesaw State University, Marietta, GA 30060, USA; [email protected] 3 Department of Physics & Astronomy, California State University, Northridge, CA 91330, USA; [email protected] * Correspondence: [email protected] † These authors contributed equally to this work. Abstract: The discovery of 3C 273 in 1963, and the emergence of the Kerr solution shortly thereafter, precipitated the current era in astrophysics focused on using black holes to explain active galactic nuclei (AGN). But while partial success was achieved in separately explaining the bright nuclei of some AGN via thin disks, as well as powerful jets with thick disks, the combination of both powerful jets in an AGN with a bright nucleus, such as in 3C 273, remained elusive. Although numerical simulations have taken center stage in the last 25 years, they have struggled to produce the conditions that explain them. This is because radiatively efficient disks have proved a challenge to simulate. Radio quasars have thus been the least understood objects in high energy astrophysics. But recent simulations have begun to change this. We explore this milestone in light of scale-invariance and show that transitory jets, possibly related to the jets seen in these recent simulations, as some have proposed, cannot explain radio quasars. We then provide a road map for a resolution. -
LIST of PUBLICATIONS Aryabhatta Research Institute of Observational Sciences ARIES (An Autonomous Scientific Research Institute
LIST OF PUBLICATIONS Aryabhatta Research Institute of Observational Sciences ARIES (An Autonomous Scientific Research Institute of Department of Science and Technology, Govt. of India) Manora Peak, Naini Tal - 263 129, India (1955−2020) ABBREVIATIONS AA: Astronomy and Astrophysics AASS: Astronomy and Astrophysics Supplement Series ACTA: Acta Astronomica AJ: Astronomical Journal ANG: Annals de Geophysique Ap. J.: Astrophysical Journal ASP: Astronomical Society of Pacific ASR: Advances in Space Research ASS: Astrophysics and Space Science AE: Atmospheric Environment ASL: Atmospheric Science Letters BA: Baltic Astronomy BAC: Bulletin Astronomical Institute of Czechoslovakia BASI: Bulletin of the Astronomical Society of India BIVS: Bulletin of the Indian Vacuum Society BNIS: Bulletin of National Institute of Sciences CJAA: Chinese Journal of Astronomy and Astrophysics CS: Current Science EPS: Earth Planets Space GRL : Geophysical Research Letters IAU: International Astronomical Union IBVS: Information Bulletin on Variable Stars IJHS: Indian Journal of History of Science IJPAP: Indian Journal of Pure and Applied Physics IJRSP: Indian Journal of Radio and Space Physics INSA: Indian National Science Academy JAA: Journal of Astrophysics and Astronomy JAMC: Journal of Applied Meterology and Climatology JATP: Journal of Atmospheric and Terrestrial Physics JBAA: Journal of British Astronomical Association JCAP: Journal of Cosmology and Astroparticle Physics JESS : Jr. of Earth System Science JGR : Journal of Geophysical Research JIGR: Journal of Indian -
Information Bulletin on Variable Stars
COMMISSIONS AND OF THE I A U INFORMATION BULLETIN ON VARIABLE STARS Nos November July EDITORS L SZABADOS K OLAH TECHNICAL EDITOR A HOLL TYPESETTING K ORI ADMINISTRATION Zs KOVARI EDITORIAL BOARD L A BALONA M BREGER E BUDDING M deGROOT E GUINAN D S HALL P HARMANEC M JERZYKIEWICZ K C LEUNG M RODONO N N SAMUS J SMAK C STERKEN Chair H BUDAPEST XI I Box HUNGARY URL httpwwwkonkolyhuIBVSIBVShtml HU ISSN COPYRIGHT NOTICE IBVS is published on b ehalf of the th and nd Commissions of the IAU by the Konkoly Observatory Budap est Hungary Individual issues could b e downloaded for scientic and educational purp oses free of charge Bibliographic information of the recent issues could b e entered to indexing sys tems No IBVS issues may b e stored in a public retrieval system in any form or by any means electronic or otherwise without the prior written p ermission of the publishers Prior written p ermission of the publishers is required for entering IBVS issues to an electronic indexing or bibliographic system to o CONTENTS C STERKEN A JONES B VOS I ZEGELAAR AM van GENDEREN M de GROOT On the Cyclicity of the S Dor Phases in AG Carinae ::::::::::::::::::::::::::::::::::::::::::::::::::: : J BOROVICKA L SAROUNOVA The Period and Lightcurve of NSV ::::::::::::::::::::::::::::::::::::::::::::::::::: :::::::::::::: W LILLER AF JONES A New Very Long Period Variable Star in Norma ::::::::::::::::::::::::::::::::::::::::::::::::::: :::::::::::::::: EA KARITSKAYA VP GORANSKIJ Unusual Fading of V Cygni Cyg X in Early November ::::::::::::::::::::::::::::::::::::::: -
Pos(MQW7)111 Ce
Long-term optical activity of the microquasar V4641 Sgr PoS(MQW7)111 Vojtechˇ Šimon Astronomical Institute, Academy of Sciences of the Czech Republic, 25165 Ondˇrejov, Czech Republic E-mail: [email protected] Arne Henden AAVSO, 49 Bay State Road, Cambridge, MA 02138, USA E-mail: [email protected] We present an analysis of the optical activity of the microquasar V4641 Sgr using the visual and photographic data. We analyze four smaller (echo) outbursts that followed the main outburst (1999). Their mean recurrence time TC is 377 days, with a trend of a decrease. We interpret the characteristic features of the recent activity of V4641 Sgr (the narrow outbursts separated by a long quiescence, a clear trend in the evolution of their TC) as the thermal instability of the accre- tion disk operating in dwarf novae and soft X-ray transients. We argue that the luminosity of four echo outbursts is too high to be explained by the thermal emission of the accretion disk. We inter- pret them as due to the thermal instability, in which the outburst gives rise to a jet radiating also in the optical. This supports the finding by Uemura et al., PASJ 54, L79 (2002). The pre-outburst observations (1964–1967) reveal ongoing activity of the system. It displays low-amplitude fluc- tuations on the timescale of several weeks, independent on the orbital phase. In addition, a larger brightening which lasted for several tens of days and occurred from the level of brightness higher than in other years can be resolved. VII Microquasar Workshop: Microquasars and Beyond September 1 - 5, 2008 Foca, Izmir, Turkey c Copyright owned by the author(s) under the terms of the Creative Commons Attribution-NonCommercial-ShareAlike Licence. -
Gamma-Ray Emission from Microquasars
UNIVERSIDAD DE BUENOS AIRES Facultad de Ciencias Exactas y Naturales Departamento de F´isica Gamma-Ray Emission from Microquasars by Mar´ia Marina Kaufman Bernad´o Supervisor: Dr. Gustavo E. Romero arXiv:astro-ph/0504498v1 22 Apr 2005 Working Place: Instituto Argentino de Radioastronom´ia (I.A.R.) Thesis Work for PhD Degree issued by the University of Buenos Aires December 15th 2004 Abstract Microquasars, X-ray binary systems that generate relativistic jets, were discovered in our Galaxy in the last decade of the XXth century. Their name indicates that they are manifestations of the same physics as quasars but on a completely different scale. Parallel to this discovery, the EGRET instrument on board of the Compton Gamma Ray Observatory detected 271 point like gamma-ray sources 170 of which were not clearly identified with known objects. This marked the beginning of gamma-ray source population studies in the Galaxy. We present in this thesis models for gamma-ray production in microquasars with the aim to propose them as possible parent populations for different groups of EGRET unidentified sources. These models are developed for a variety of scenarios taking into account several possible combinations, i.e. black holes or neutron stars as the compact object, low mass or high mass stellar companions, as well as leptonic or hadronic gamma-ray production processes. We also show that the presented models for gamma-rays emitting microquasars can be used to explain observations from well known sources that are detected in energy ranges other than EGRET’s. Finally, we include an alternative gamma-ray producing situation that does not involve microquasars but a specific unidentified EGRET source possibly linked to a magnetized accreting pulsar. -
Infrared Study of H1743-322 in Outburst: a Radio-Quiet and NIR-Dim
Astronomy & Astrophysics manuscript no. h1743˙vsubm-final c ESO 2021 June 13, 2021 Infrared study of H 1743-322 in outburst: a radio-quiet and NIR-dim microquasar⋆ S. Chaty1,2, A.J. Mu˜noz Arjonilla3, and G. Dubus4,5 1 AIM (UMR-E 9005 CEA/DSM-CNRS-Universit´eParis Diderot), Irfu/Service d’Astrophysique, Centre de Saclay, FR-91191 Gif- sur-Yvette Cedex, France, e-mail: [email protected] 2 Institut Universitaire de France, 103, boulevard Saint-Michel 75005 Paris, France 3 Grupo de Investigaci´on FQM-322, Universidad de Ja´en, Campus Las Lagunillas s/n A3-065, E-23071 - Ja´en, Spain, e-mail: [email protected] 4 Univ. Grenoble Alpes, IPAG, F-38000 Grenoble, France, e-mail: [email protected] 5 CNRS, IPAG, F-38000 Grenoble, France Received March 15, 2013; accepted April 15, 2013 ABSTRACT Context. Microquasars are accreting Galactic sources that are commonly observed to launch relativistic jets. One of the most important issues regarding these sources is the energy budget of ejections relative to the accretion of matter. Aims. The X-ray binary, black hole candidate, and microquasar H 1743-322 exhibited a series of X-ray outbursts between 2003 and 2008. We took optical and near-infrared (OIR) observations with the ESO/NTT telescope during three of these outbursts (2003, 2004, and 2008). The goals of these observations were to investigate the presence of a jet, and to disentangle the various contributions constituting the spectral energy distribution (SED): accretion, ejection, and stellar emission. Methods. Photometric and spectroscopic OIR observations allowed us to produce a high time-resolution lightcurve in Ks-band, to analyze emission lines present in the IR spectra, to construct a multiwavelength SED including radio, IR, and X-ray data, and to complete the OIR vs X-ray correlation of black hole binaries with H 1743-322 data points. -
Modified Viscosity in Accretion Disks. Application to Galactic Black Hole
Astronomy & Astrophysics manuscript no. languagecorrection_doarxiva c ESO 2017 April 24, 2017 Modified viscosity in accretion disks. Application to Galactic black hole binaries, intermediate mass black holes, and AGN Mikołaj Grz˛edzielski1, Agnieszka Janiuk1, Bo˙zenaCzerny1 and Qingwen Wu2 1 Center for Theoretical Physics, Polish Academy of Sciences, Al. Lotnikow 32/46, 02-668 Warsaw, Poland e-mail: [email protected] 2 School of Physics, Huazhong University of Science and Technology, Wuhan 430074, China Received ...; accepted ... ABSTRACT Aims Black holes (BHs) surrounded by accretion disks are present in the Universe at different scales of masses, from microquasars up to the active galactic nuclei (AGNs). Since the work of Shakura and Sunyaev (1973) and their α-disk model, various prescriptions for the heat-production rate are used to describe the accretion process. The current picture remains ad hoc due the complexity of the magnetic field action. In addition, accretion disks at high Eddington rates can be radiation-pressure dominated and, according to some of the heating prescriptions, thermally unstable. The observational verification of their resulting variability patterns may shed light on both the role of radiation pressure and magnetic fields in the accretion process. Methods We compute the structure and time evolution of an accretion disk, using the code GLADIS (which models the global accretion disk instability). We supplement this model with a modified viscosity prescription, which can to some extent describe the magnetisation of the disk. We study the results for a large grid of models, to cover the whole parameter space, and we derive conclusions separately for different scales of black hole masses, which are characteristic for various types of cosmic sources. -
Observational Evidence for Tidal Interaction in Close Binary Systems, Including Short-Period Extrasolar Planets
Tidal effects in stars, planets and disks M.-J. Goupil and J.-P. Zahn EAS Publications Series, Vol. ?, 2018 OBSERVATIONAL EVIDENCE FOR TIDAL INTERACTION IN CLOSE BINARY SYSTEMS Tsevi Mazeh1 Abstract. This paper reviews the rich corpus of observational evidence for tidal effects, mostly based on photometric and radial-velocity mea- surements. This is done in a period when the study of binaries is being revolutionized by large-scaled photometric surveys that are detecting many thousands of new binaries and tens of extrasolar planets. We begin by examining the short-term effects, such as ellipsoidal variability and apsidal motion. We next turn to the long-term effects, of which circularization was studied the most: a transition period be- tween circular and eccentric orbits has been derived for eight coeval samples of binaries. The study of synchronization and spin-orbit align- ment is less advanced. As binaries are supposed to reach synchroniza- tion before circularization, one can expect finding eccentric binaries in pseudo-synchronization state, the evidence for which is reviewed. We also discuss synchronization in PMS and young stars, and compare the emerging timescale with the circularization timescale. We next examine the tidal interaction in close binaries that are orbited by a third distant companion, and review the effect of pumping the binary eccentricity by the third star. We elaborate on the impact of the pumped eccentricity on the tidal evolution of close binaries residing in triple systems, which may shrink the binary separation. Finally we consider the extrasolar planets and the observational evidence for tidal interaction with their parent stars.