Improving Water Use Efficiency by Reducing Groundwater Recharge Under Irrigated Pastures
Total Page:16
File Type:pdf, Size:1020Kb
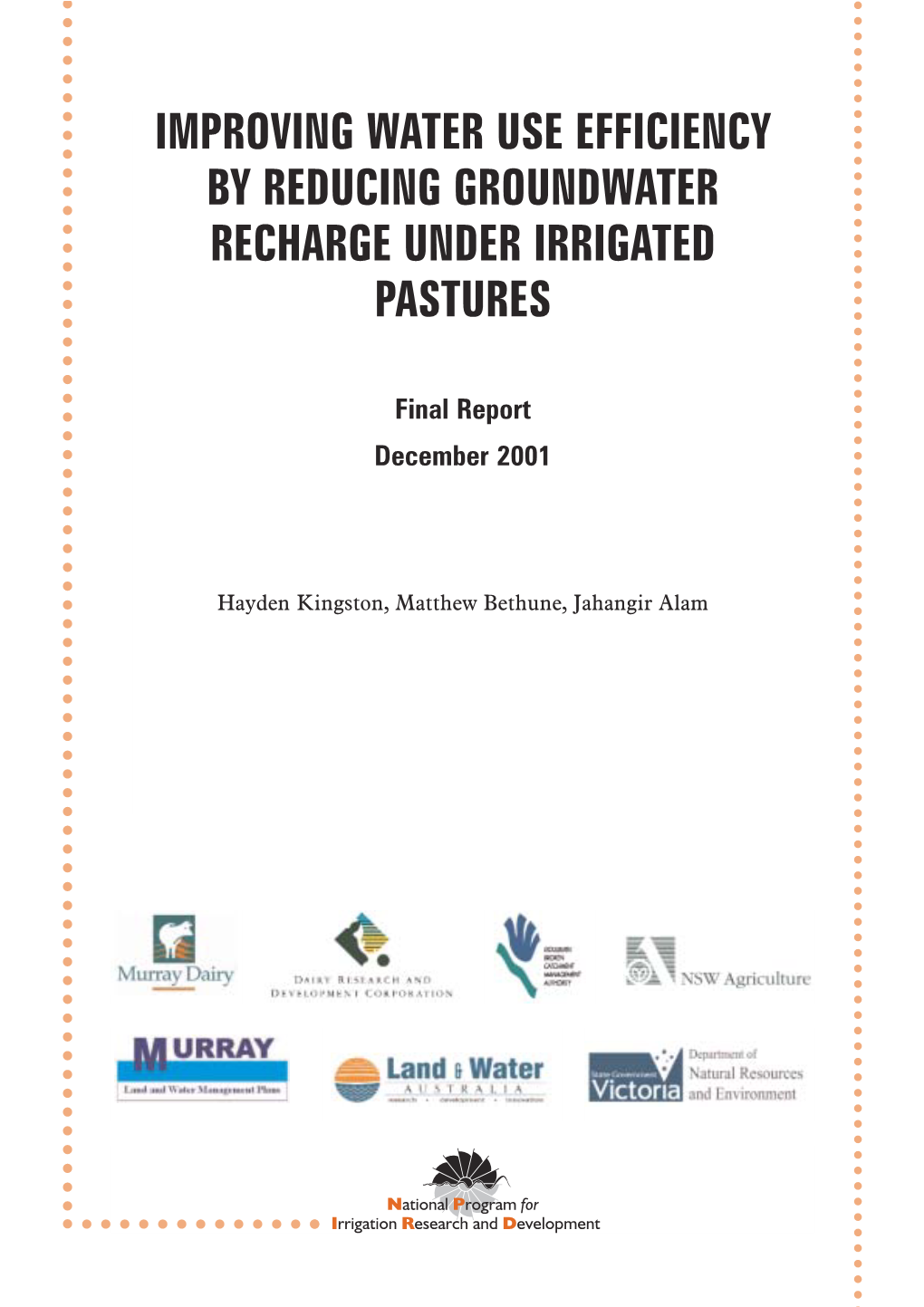
Load more
Recommended publications
-
Forty -Second Annual Report
1955 VICTORIA COUNTRY ROADS BOARD FORTY -SECOND ANNUAL REPORT FOR YEAR ENDED 30TH JUNE, 1955 PRESENTED TO BOT:S. HOUSES 01<' PARLIAMENT PURSUA~'l' ro ACT No. 3662. ! Approximate Cost ot Rttport. ~ Preparat10tl1 nor. given. Printiug t l,OfJH l.:'oJ.Ue&J, ~770 ] By Autbomy : \V. J\L HOUSTON. c;OVERNMENT PRINTER. MELBOURNE. ;'i<o. 32.-[3s. 6d.i-l2648;55. Dual carriageway and channelization of Princes Highway through township of Dandenong. Cover.-A recently constructed pre-cast concrete bridge over Lightning Creek on tne Omeo Hignway. COUNTRY ROADS BOARD FORTY-SECOND ANNUAL REPORT, 1955 CONTENTS FINANCE~ Page Increase in revenue 7 fn&dequacy of funds for present works 9 Applications for funds 9 Receipts from motor registration fees, &c. 10 Commonwealth Aid Roads Act 1954 .. 10 I.nan moneys 11 Total works, allocation 11 MAIN ROADS- Allocation of funds 11 Apportionment of costs 11 Contributions by Municipal Councils 12 Particulars of works carried out 12 STATE HIGHWAYS- Restricted allocation of funds 18 Works carried out 18 TOURISTS' ROADS- Allocation of funds 27 Works carried out 27 J<'ORESTS RoADS- Funds allocated 29 Works carried out 29 UNCLASSIFIED ROADS- Applications from Councils for grants 29 Amounts allotted 29 Major works cal'ried out 29 BRIDGES- Board's financial resources immfficient 32 Construction-completed or in progress 32 Metropolitan bridges as Country bridges .. 33 J!'LOOD AND RGSH FIRE DAMAGE-- Most seriously affected areas 43 Applications received and grants made 43 WORKS FOR OTiillR AuTHORITms Department of Public Works -
Electric Light and Power Act 1896
519 I Hl5 VICTORIA. REPO rtT RK8PitCTlNO APPLICA~TIONS ANI) PROCEEDING~ t:NDKR THK ELECTRIC LIGHT AND POWER ACT 1896 J.<~OI~ THE YEAI(; 1f)l4. PRESENTED TO BOTH HOUSES OF PARLIAMENT PURSUANT TO LAW ~)1 6\nthDtitt : ALTIRRT J. MtTLLETT, GOVERNMF.ST PRI!Ii'I'KR, MELTIO{lllNJt. So. 20 [ls.]-8i74 APPROXIMATE COST OF REPORT. £, •• d. Preparatio t-NoL ghen. Printing (550 copies) •• 1S 0 0 521 REP 0 RT. The following Report for the year ended 31st December, 1914, is presented to Parliament in accordance with the provisions of Section 57 of the Electt·ic Light and Power Act 1896, No. 1413 :- LIST OF APPLICATIONS FOR ORDERS TO AUTHORIZE THE SUPPLY OF ELECTRICITY PLACED BEFORE THE MINISTER OF PUBLIC WORKS DURING THE YEAR ENDED 31ST DECEMBER, 1914.. N ~me of Applicant. Proposed Area of Supply. ···-·-------··-----1 ~---··--·-------------- Cock's Pioneer Gold an<l Tin Mines No }Jortion of the Shire of Xorth Ovens. Liability. The Conncil of the ~hire of Hei•lelberg 'l'he Fairfield, Ivanhoe, and Heidelberg Hidings of the Shire. The Council of the Shire of W errihee Portion of the Shire of W erribee. The Council of the Shire of Lilvdalc T!Je South-west Riding of the Shire of Lilydale. The Melbourne Electric Supply. Co. Ltd. Portion of the Shire of South Barwon. The Council of the Town ol' Coburg The Town of Coburg. The South Dandenong Electric Light Portion of the South Riding of the Shire of Dandenong, Svndicale The· Council of the Shire of Lancefield The Shire of Lancefield. The Council of the Shire of Doncaster The ~hire of Doncaster. -
GREATER SHEPPARTON DRAFT HERITAGE STRATEGY 2019 Sculpture and Standing Stone Commemorating Joseph Furphy, Author of ‘Such Is Life’ (1903), Shepparton
GREATER SHEPPARTON DRAFT HERITAGE STRATEGY 2019 Sculpture and standing stone commemorating Joseph Furphy, author of ‘Such is Life’ (1903), Shepparton Draft Greater Shepparton Heritage Strategy 2019 2 Contents Acknowledgements .................................................................................................. 4 Glossary of terms ..................................................................................................... 4 Valuing our heritage............................................................................................ 5 Council’s Commitment to our Heritage ............................................................... 5 Introduction ........................................................................................................ 6 What is Heritage? .................................................................................................... 6 Approaching Heritage Conservation ........................................................................... 6 Understanding cultural significance............................................................................ 7 Council’s Role .......................................................................................................... 7 About the Heritage Strategy ..................................................................................... 8 Part 1: Background ............................................................................................. 9 1.1 Present City of Greater Shepparton ..................................................................... -
Economic Development Strategy
Economic Development Strategy 2014-2019 Disclaimer: The Shire of Campaspe Economic Whilst all care and diligence have been exercised in the preparation of this report, Development Strategy 2014–2019 AEC Group Pty Ltd does not warrant the accuracy of the information contained within was funded through a partnership with and accepts no liability for any loss or damage that may be suffered as a result of the Victorian State Government and reliance on this information, whether or not there has been any error, omission or the Shire of Campaspe. AEC Group negligence on the part of AEC Group Pty Ltd or their employees. Any forecasts or Pty Ltd was engaged to develop the projections used in the analysis can be affected by a number of unforeseen variables, Economic Development Strategy. and as such no warranty is given that a particular set of results will in fact be achieved. Shire of Campaspe g i Economic Development Strategy 2014-2019 EXECUTIVE Table ES.1: Campaspe Key Socio-Economic Indicators SUMMARY Extensive research and consultation has been undertaken in developing the direction and content of the Shire of Campaspe Economic Development Strategy (the Strategy). The Strategy will guide and direct Council’s role in implementing immediate Key Statistics Period Indicator 5-Year economic development priorities and day-to-day Change tasks to assist in promoting the Shire, attracting Population (no.) 2013 36,919 - 0.3% investment and supporting business and industry Labour force (no.) Dec-13 20,376 14.6% growth. Unemployment rate (%) Dec-13 4.6% 0.4% The Shire of Campaspe is a vibrant rural community in northern Gross Regional Product 2012-13 $1,930.3 5.6% Victoria comprising many townships such as Echuca, Kyabram, ($’M) Rochester, Lockington, Gunbower, Rushworth, Stanhope, Top 3 Sectors by Industry Value Add Girgarre, Toolleen and Tongala. -
SCG Victorian Councils Post Amalgamation
Analysis of Victorian Councils Post Amalgamation September 2019 spence-consulting.com Spence Consulting 2 Analysis of Victorian Councils Post Amalgamation Analysis by Gavin Mahoney, September 2019 It’s been over 20 years since the historic Victorian Council amalgamations that saw the sacking of 1600 elected Councillors, the elimination of 210 Councils and the creation of 78 new Councils through an amalgamation process with each new entity being governed by State appointed Commissioners. The Borough of Queenscliffe went through the process unchanged and the Rural City of Benalla and the Shire of Mansfield after initially being amalgamated into the Shire of Delatite came into existence in 2002. A new City of Sunbury was proposed to be created from part of the City of Hume after the 2016 Council elections, but this was abandoned by the Victorian Government in October 2015. The amalgamation process and in particular the sacking of a democratically elected Council was referred to by some as revolutionary whilst regarded as a massacre by others. On the sacking of the Melbourne City Council, Cr Tim Costello, Mayor of St Kilda in 1993 said “ I personally think it’s a drastic and savage thing to sack a democratically elected Council. Before any such move is undertaken, there should be questions asked of what the real point of sacking them is”. Whilst Cr Liana Thompson Mayor of Port Melbourne at the time logically observed that “As an immutable principle, local government should be democratic like other forms of government and, therefore the State Government should not be able to dismiss any local Council without a ratepayers’ referendum. -
Shepparton City Council Heritage Study Stage IIB Tatura Contextual History Prepared By: Heritage Concepts
Volume 2: Greater Shepparton City Council Heritage Study Stage IIB Tatura Contextual History Prepared by: Heritage Concepts JULY 2010 Tatura: A Contextual History 2010 Greater Shepparton Heritage Study Stage IIB The following history contains a summary of pertinent historic themes and descriptions of events that have informed the selection of items of cultural heritage significance for the Greater Shepparton Heritage Study Stage IIB. Memorial Clock Tatura Page 2 of 23 Tatura: A Contextual History 2010 Greater Shepparton Heritage Study Stage IIB TABLE OF CONTENTS 1.0 Introduction ................................................................................................................... 6 2.0 Agricultural Activities, Settlement and Township Building ...................................... 7 2.1 Squatting era, the Winter family and Dhurringile .................................................................... 7 2.2 Land Selection and Settlement ................................................................................................ 8 3.0 The Development of Tatura ......................................................................................... 8 3.1 The Whim ............................................................................................................................... 8 3.2 Tatura 1874 – 1885 ................................................................................................................. 9 3.3 Tatura 1886 – 1898 .............................................................................................................. -
Newsletter 42 Web.Pub
Newsletter No 42 October 2011 SHEPPARTON FAMILY HISTORY GROUP inc Shepparton Family History Group report Since our last newsletter Shepparton Family History Group members, capably led by Kerry, have completed the checking of nearly 11,000 photographic images taken of the Rodney Shire rate books as well as re- photographing any pages which were not of acceptable quality. The massive task of indexing all the names appearing in the rate books, covering the years 1886 to 1952, has been commenced by one of our members. Fay is no stranger to the tedious computer work involved with indexing having undertaken many other similar tasks for our group. The rate book copies are available to view on one the computers in our room but at present they will have to be searched by individual years and individual shire ridings. The New Zealand research day we held during family history week was a great success. Our member with New Zealand links, Jenny Baldwin, conducted the seminar and had all in attendance very happy with her com- plete roundup of possible places and web sites to track down any family history information relating to New Zealand (more details on page 2). Our annual meeting was conducted in July, all the serving office bearers were returned (details on page 8). Following the success of our two earlier books, the Shepparton Family History Group intends to publish Book 3 of “Early Families of Shepparton and District ” Was your family in Shepparton and district before 1940? Would you like your family included? Write an account of no more than 1,000 words. -
Local Government (Validation) Act 1988 No
Local Government (Validation) Act 1988 No. 71 of 1988 TABLE OF PROVISIONS Section 1. Purpose. 2. Commencement. 3. Validation of Orders in Council. 4. Shire of Kyneton. 5. Shire of Colac and Dimboola. 6. Review of internal boundaries. THE SCHEDULE 1177 Victoria No. 71 of 1988 Local Government (Validation) Act 1988 [Assented to 15 December 1988] The Parliament of Victoria enacts as follows: Purpose. 1. The purpose of this Act is to validate certain Orders made under Part II of the Local Government Act 1958 and for certain other purposes. Commencement. 2. This Act comes into operation on the day on which it receives the Royal Assent. Validation of Orders in Council. 3. (1) An Order made by the Governor in Council under Part II of the Local Government Act 1958 in relation to a municipality referred to in column 1 of an item in the Schedule and published in the Government Gazette on the date referred to in column 3 of that item shall be deemed to have taken effect in accordance with that Part on the date referred to in column 4 of that item and thereafter always to have been valid. 1179. s. 4 Local Government (Validation) Act 1988 (2) Any election for councillors of a municipality referred to in an item in the Schedule, and any thing done by or in relation to that municipality or its Council or persons acting as its councillors or otherwise affecting that municipality, on or after the date on which the Order referred to in that item took effect shall be deemed to have been as validly held or done as it would have been if sub-section (1) had been in force on that date. -
Electric Light and Power Act 1896
397 1913. VICTORIA. RESPECTING APPLICATIONS AND PHOCEE DI NUS UNDER THE ELECTRIC LIGHT AND POWER ACT 1896 F(>R THE YEA H. 1912. @)} ~nt1t.orit11: ALBRR'l' J. MULLE'l'T, GOVERNMENT PR.I.NTU, MELBOUUJL No. 29.-fls.] -8880. APPROXIMATE COST OF REPORT. £, •• d. Prepar,.tio"-··Not gh·en. Printin~ (500 copieo) •• 16 0 0 399 REP 0 RT. The following Report for the year ended 31st Decem her, 1912, is presented to Parliameut in accordance with the provisions of Section 57 of the Elect1-ic Light find Puwer Act 18~6, No. 1413 :- LIST OF APPLICATIONS FOR ORDERS TO AUTHORIZE THE SUPPLY OF ELECTRICITY PLACED BEFORE THE l\1INISTER OF PUBLIC WORKS DURING THE YEAR ENDED 31ST DECEMBER, 1912. :"nmr of Applicant. Area of Supply. The Council of the Borough of Koroit The municipal district of the Borough of Koroit The Council of the Shire of Kar karooc The Township of Hopetoun The Council of the Shire of Kar karooc The Township ol Bt>ulah The Council of the Citv of Brun,wick The municipal distrid ol' the City of Brunswick The Council of the Shire of LHatfra 'I'he Township of .Maffra, and certain porrious <>lllside its boundaries The Conncil of the Borough of H~rsham The municipal district of the Borough of Horsham The Council of the Shire of Yea ••. The Township of Yea and certain portions outside its bnund11.riPs The Council of the Shire of Nnnawading The mrrnidpal district of tbe Shire of Nunawading The Counl'il of the Shire of Mcl vor The Town~hrp of Heath1·ote The Corncil uf the Borough of WonthRggi .. -
Greater Shepparton Heritage Study Stage IIC 2017
GREATER SHEPPARTON HERITAGE STUDY STAGE IIC Prepared for Greater Shepparton City Council By Heritage Concepts Pty Ltd June 2017 GREATER SHEPPARTON HERITAGE STUDY (Stage IIC) 2017 Project Team Deborah Kemp, Heritage Concepts Pty Ltd Greater Shepparton Heritage Advisory Committee Local heritage makes the greatest contribution to forming our living historic environment, more so than the small number of outstanding items of state, national or world significance. Greater than the sum of its parts, the varied collection of local heritage in an area enriches its character and gives identity to a neighbourhood, region or town in a way that cannot be reproduced. Local heritage is often what makes an area distinctive in the long–term, even if the heritage features were once in a neglected state or considered unremarkable… (Heritage Council of NSW, Levels of Heritage Significance, 2008) 1 GREATER SHEPPARTON HERITAGE STUDY (Stage IIC) 2017 CONTENTS Acknowledgements Executive Statement 3 1.0 Introduction 5 2.0 Purpose 6 3.0 Selection Process 8 4.0 Methodology 9 5.0 Thresholds of Significance 10 5.1 Thematic Environmental History 12 5.2 Other factors to be considered when assessing significance 12 6.0 The Statement of Significance 13 7.0 Recommendations 13 8.0 List of Places 18 APPENDIX A HERCON model criteria 31 APPENDIX B HERMES citations 34 2 GREATER SHEPPARTON HERITAGE STUDY (Stage IIC) 2017 Executive Statement This report describes the key tasks and the methodology for the Greater Shepparton Heritage Study Stage IIC and the conclusions and recommendations that have arisen from its completion. The purpose of this Study is to document places of post contact cultural heritage significance to the City of Greater Shepparton and to make recommendations for their conservation. -
City of Greater Shepparton Heritage Study Stage Ii
CITY OF GREATER SHEPPARTON HERITAGE STUDY STAGE II HERITAGE PLACE DATASHEETS The Echoes (HO11) VOLUME 3 DATASHEETS A-Mooroopna CITY OF GREATER SHEPPARTON HERITAGE STUDY STAGE 11 HERITAGE PLACE DATASHEETS VOLUME 3 DATASHEETS A-Mooroopna Allom Lovell & Associates Conservation Architects 35 Little Bourke Street Melbourne 3000 February 2004 This report is Volume 3 of a six-volume set, comprising: Volume 1 Introduction and Recommendations Volume 2 Environmental History Volume 3 Heritage Place Datasheets: A-Mooroopna Volume 4 Heritage Place Datasheets: Murchison –Z Volume 5 Heritage Overlay Precincts Volume 6 Heritage Place Datasheets: Log Structures and Scotch Kiln City of Greater Shepparton Heritage Study Stage Two DATASHEETS A-MOOROOPNA Allom Lovell & Associates 5 City of Greater Shepparton Heritage Study Stage II 6 Allom Lovell & Associates City of Greater Shepparton Heritage Study Stage Two Current Name Holy Trinity Anglican Church Reference No. 01 (HO1) Former Name Grade B Address Lenne Road, Ardmona Construction Date 1914-15 Map Reference 471.722 Shepparton Ownership Anglican Church Survey Date August 2002 Intactness Condition 9 Good Fair Poor 9 Good Fair Poor Heritage Listings Existing Existing Recommended VHR RNE Planning Scheme 9 National Trust (Vic) Allom Lovell & Associates 7 City of Greater Shepparton Heritage Study Stage Two History Prior to 1914, the Anglican congregation at Ardmona had gathered in the local public hall for services. Half an acre of orchard land was donated by a local farmer, Mr Hornidge, as the site for a new church. The foundation stone for the Holy Trinity Anglican Church was laid on 9 December 1914 by Bishop J D Langley. -
El Ectric Light a Nd Po,Ver Act 1896
1914. VICTORIA. -- --------------------------------------------- RESPECTING APPLICA~riOXS ANI> PROCEEl)INGS UNDER THE EL_ECTRIC LIGHT _A_ND PO,VER ACT 1896 FOR rrHE YEAR 1913. ~JJ .;tutltority: .&.LDERT J, MULLETT, GOVER:SJ\IENT PRINTER, MELBOURN:l!:, Al'PRUXIMATE COST OF REl'ORT. £ •. d. Preparati•rr-·Not~ g-i·•en. Pl·iiHin!.{ (500 coples) n 11 0 0 '~ ....... -... REPOR1\ The following Report for the year ended 3lst Decem her, 19 U1, is presented to Parliament in accordance with the provisions of Section 57 of the Electric Ligltt rmd Power Act 1896, No. l413 :- LIST OF APPLICATI0~3 FOR ORDERS 1'0 AUTHOR[ZE THE SUPPLY OB' ELECTRICITY PLACED BEFORE THE Mr~ISTER. OB' PUBLIC WORKS DURING THE YEAR ENDED 31ST DECEMBER, 1913. !\arua o! Applicant. Proposed Area of Supply. --·-····-~··-···~~-· -~~-~~~~----- The Conncil of the Shire of Gortlon Portion of the municipal district of the Hhire of Gordon The Council of the Shire of .Milunra Pot"tion of the municipal district of the Shi1 e of 1\hldum The Producial Elec:rie Development Co. Portion of the municipal district of the Borough of Mary- borough The India Rubber, Gutta Percha and Tele- The municipal district of the Township of Colac graph Works Co. Ltd. • The Council of the Shire of Nnmnrkah The municipal district of the Towuship of 'N at.halia. The Council of the ~l1ire of Ito.lney Portion of the rnnnicipal district of the Shire of Rodney, including the Tow'nship of .Mooroopna. The Council of the Shire of Gisborne Portion of the municipal district of t.he Shire of Gisborne The Council of the !::\hire of Dimboola Porilon of the municipal district of the Shire of Dirnlloola ORDERS GRANTED DURING THE YEAR 1913.