Nasa Tn D-3220 Efficient Planetary Parking Orbits
Total Page:16
File Type:pdf, Size:1020Kb
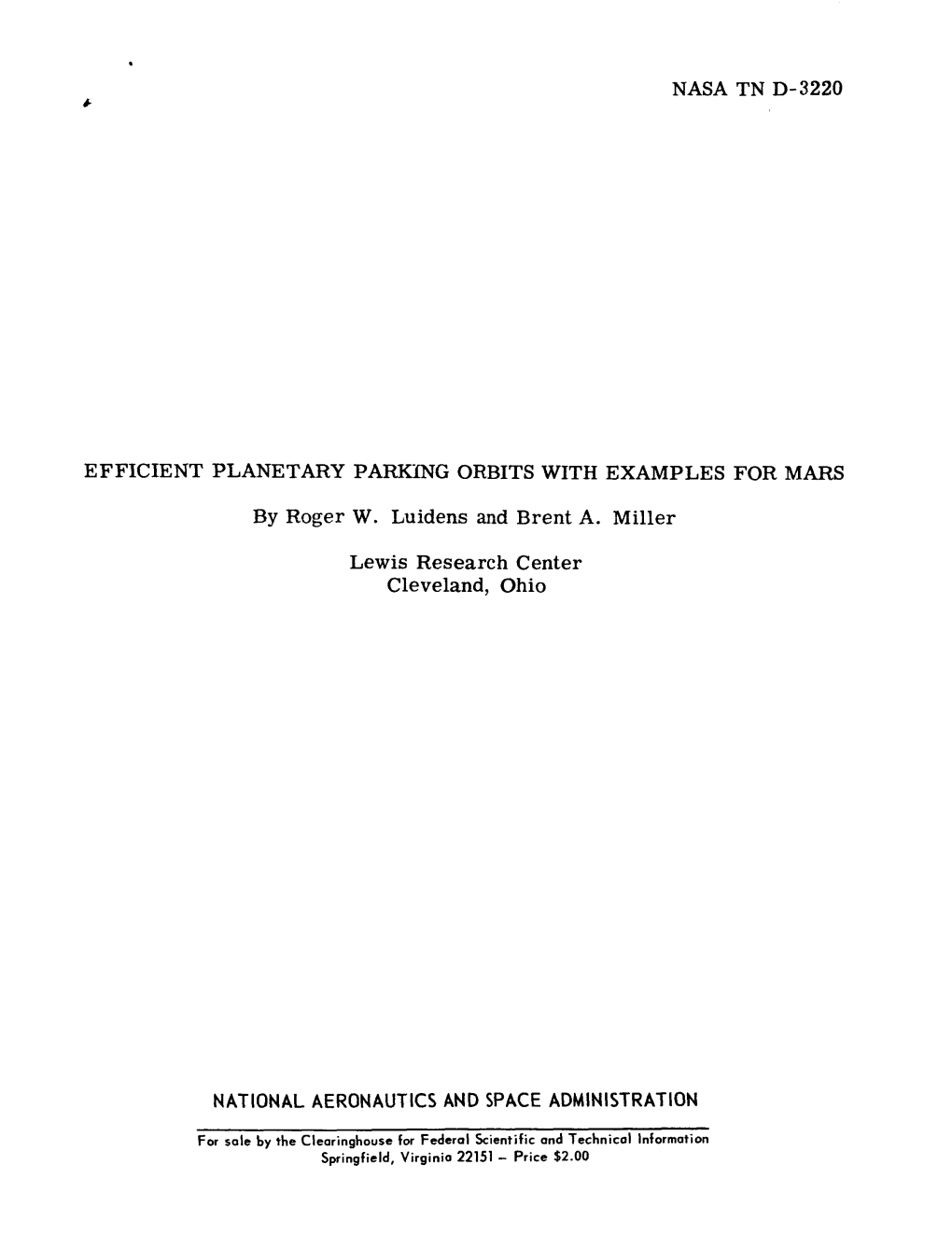
Load more
Recommended publications
-
Astrodynamics
Politecnico di Torino SEEDS SpacE Exploration and Development Systems Astrodynamics II Edition 2006 - 07 - Ver. 2.0.1 Author: Guido Colasurdo Dipartimento di Energetica Teacher: Giulio Avanzini Dipartimento di Ingegneria Aeronautica e Spaziale e-mail: [email protected] Contents 1 Two–Body Orbital Mechanics 1 1.1 BirthofAstrodynamics: Kepler’sLaws. ......... 1 1.2 Newton’sLawsofMotion ............................ ... 2 1.3 Newton’s Law of Universal Gravitation . ......... 3 1.4 The n–BodyProblem ................................. 4 1.5 Equation of Motion in the Two-Body Problem . ....... 5 1.6 PotentialEnergy ................................. ... 6 1.7 ConstantsoftheMotion . .. .. .. .. .. .. .. .. .... 7 1.8 TrajectoryEquation .............................. .... 8 1.9 ConicSections ................................... 8 1.10 Relating Energy and Semi-major Axis . ........ 9 2 Two-Dimensional Analysis of Motion 11 2.1 ReferenceFrames................................. 11 2.2 Velocity and acceleration components . ......... 12 2.3 First-Order Scalar Equations of Motion . ......... 12 2.4 PerifocalReferenceFrame . ...... 13 2.5 FlightPathAngle ................................. 14 2.6 EllipticalOrbits................................ ..... 15 2.6.1 Geometry of an Elliptical Orbit . ..... 15 2.6.2 Period of an Elliptical Orbit . ..... 16 2.7 Time–of–Flight on the Elliptical Orbit . .......... 16 2.8 Extensiontohyperbolaandparabola. ........ 18 2.9 Circular and Escape Velocity, Hyperbolic Excess Speed . .............. 18 2.10 CosmicVelocities -
A Hot Subdwarf-White Dwarf Super-Chandrasekhar Candidate
A hot subdwarf–white dwarf super-Chandrasekhar candidate supernova Ia progenitor Ingrid Pelisoli1,2*, P. Neunteufel3, S. Geier1, T. Kupfer4,5, U. Heber6, A. Irrgang6, D. Schneider6, A. Bastian1, J. van Roestel7, V. Schaffenroth1, and B. N. Barlow8 1Institut fur¨ Physik und Astronomie, Universitat¨ Potsdam, Haus 28, Karl-Liebknecht-Str. 24/25, D-14476 Potsdam-Golm, Germany 2Department of Physics, University of Warwick, Coventry, CV4 7AL, UK 3Max Planck Institut fur¨ Astrophysik, Karl-Schwarzschild-Straße 1, 85748 Garching bei Munchen¨ 4Kavli Institute for Theoretical Physics, University of California, Santa Barbara, CA 93106, USA 5Texas Tech University, Department of Physics & Astronomy, Box 41051, 79409, Lubbock, TX, USA 6Dr. Karl Remeis-Observatory & ECAP, Astronomical Institute, Friedrich-Alexander University Erlangen-Nuremberg (FAU), Sternwartstr. 7, 96049 Bamberg, Germany 7Division of Physics, Mathematics and Astronomy, California Institute of Technology, Pasadena, CA 91125, USA 8Department of Physics and Astronomy, High Point University, High Point, NC 27268, USA *[email protected] ABSTRACT Supernova Ia are bright explosive events that can be used to estimate cosmological distances, allowing us to study the expansion of the Universe. They are understood to result from a thermonuclear detonation in a white dwarf that formed from the exhausted core of a star more massive than the Sun. However, the possible progenitor channels leading to an explosion are a long-standing debate, limiting the precision and accuracy of supernova Ia as distance indicators. Here we present HD 265435, a binary system with an orbital period of less than a hundred minutes, consisting of a white dwarf and a hot subdwarf — a stripped core-helium burning star. -
Electric Propulsion System Scaling for Asteroid Capture-And-Return Missions
Electric propulsion system scaling for asteroid capture-and-return missions Justin M. Little⇤ and Edgar Y. Choueiri† Electric Propulsion and Plasma Dynamics Laboratory, Princeton University, Princeton, NJ, 08544 The requirements for an electric propulsion system needed to maximize the return mass of asteroid capture-and-return (ACR) missions are investigated in detail. An analytical model is presented for the mission time and mass balance of an ACR mission based on the propellant requirements of each mission phase. Edelbaum’s approximation is used for the Earth-escape phase. The asteroid rendezvous and return phases of the mission are modeled as a low-thrust optimal control problem with a lunar assist. The numerical solution to this problem is used to derive scaling laws for the propellant requirements based on the maneuver time, asteroid orbit, and propulsion system parameters. Constraining the rendezvous and return phases by the synodic period of the target asteroid, a semi- empirical equation is obtained for the optimum specific impulse and power supply. It was found analytically that the optimum power supply is one such that the mass of the propulsion system and power supply are approximately equal to the total mass of propellant used during the entire mission. Finally, it is shown that ACR missions, in general, are optimized using propulsion systems capable of processing 100 kW – 1 MW of power with specific impulses in the range 5,000 – 10,000 s, and have the potential to return asteroids on the order of 103 104 tons. − Nomenclature -
AFSPC-CO TERMINOLOGY Revised: 12 Jan 2019
AFSPC-CO TERMINOLOGY Revised: 12 Jan 2019 Term Description AEHF Advanced Extremely High Frequency AFB / AFS Air Force Base / Air Force Station AOC Air Operations Center AOI Area of Interest The point in the orbit of a heavenly body, specifically the moon, or of a man-made satellite Apogee at which it is farthest from the earth. Even CAP rockets experience apogee. Either of two points in an eccentric orbit, one (higher apsis) farthest from the center of Apsis attraction, the other (lower apsis) nearest to the center of attraction Argument of Perigee the angle in a satellites' orbit plane that is measured from the Ascending Node to the (ω) perigee along the satellite direction of travel CGO Company Grade Officer CLV Calculated Load Value, Crew Launch Vehicle COP Common Operating Picture DCO Defensive Cyber Operations DHS Department of Homeland Security DoD Department of Defense DOP Dilution of Precision Defense Satellite Communications Systems - wideband communications spacecraft for DSCS the USAF DSP Defense Satellite Program or Defense Support Program - "Eyes in the Sky" EHF Extremely High Frequency (30-300 GHz; 1mm-1cm) ELF Extremely Low Frequency (3-30 Hz; 100,000km-10,000km) EMS Electromagnetic Spectrum Equitorial Plane the plane passing through the equator EWR Early Warning Radar and Electromagnetic Wave Resistivity GBR Ground-Based Radar and Global Broadband Roaming GBS Global Broadcast Service GEO Geosynchronous Earth Orbit or Geostationary Orbit ( ~22,300 miles above Earth) GEODSS Ground-Based Electro-Optical Deep Space Surveillance -
The Velocity Dispersion Profile of the Remote Dwarf Spheroidal Galaxy
The Velocity Dispersion Profile of the Remote Dwarf Spheroidal Galaxy Leo I: A Tidal Hit and Run? Mario Mateo1, Edward W. Olszewski2, and Matthew G. Walker3 ABSTRACT We present new kinematic results for a sample of 387 stars located in and around the Milky Way satellite dwarf spheroidal galaxy Leo I. These spectra were obtained with the Hectochelle multi-object echelle spectrograph on the MMT, and cover the MgI/Mgb lines at about 5200A.˚ Based on 297 repeat measure- ments of 108 stars, we estimate the mean velocity error (1σ) of our sample to be 2.4 km/s, with a systematic error of 1 km/s. Combined with earlier re- ≤ sults, we identify a final sample of 328 Leo I red giant members, from which we measure a mean heliocentric radial velocity of 282.9 0.5 km/s, and a mean ± radial velocity dispersion of 9.2 0.4 km/s for Leo I. The dispersion profile of ± Leo I is essentially flat from the center of the galaxy to beyond its classical ‘tidal’ radius, a result that is unaffected by contamination from field stars or binaries within our kinematic sample. We have fit the profile to a variety of equilibrium dynamical models and can strongly rule out models where mass follows light. Two-component Sersic+NFW models with tangentially anisotropic velocity dis- tributions fit the dispersion profile well, with isotropic models ruled out at a 95% confidence level. Within the projected radius sampled by our data ( 1040 pc), ∼ the mass and V-band mass-to-light ratio of Leo I estimated from equilibrium 7 models are in the ranges 5-7 10 M⊙ and 9-14 (solar units), respectively. -
Use of MESSENGER Radioscience Data to Improve Planetary Ephemeris and to Test General Relativity
A&A 561, A115 (2014) Astronomy DOI: 10.1051/0004-6361/201322124 & © ESO 2014 Astrophysics Use of MESSENGER radioscience data to improve planetary ephemeris and to test general relativity A. K. Verma1;2, A. Fienga3;4, J. Laskar4, H. Manche4, and M. Gastineau4 1 Observatoire de Besançon, UTINAM-CNRS UMR6213, 41bis avenue de l’Observatoire, 25000 Besançon, France e-mail: [email protected] 2 Centre National d’Études Spatiales, 18 avenue Édouard Belin, 31400 Toulouse, France 3 Observatoire de la Côte d’Azur, GéoAzur-CNRS UMR7329, 250 avenue Albert Einstein, 06560 Valbonne, France 4 Astronomie et Systèmes Dynamiques, IMCCE-CNRS UMR8028, 77 Av. Denfert-Rochereau, 75014 Paris, France Received 24 June 2013 / Accepted 7 November 2013 ABSTRACT The current knowledge of Mercury’s orbit has mainly been gained by direct radar ranging obtained from the 60s to 1998 and by five Mercury flybys made with Mariner 10 in the 70s, and with MESSENGER made in 2008 and 2009. On March 18, 2011, MESSENGER became the first spacecraft to orbit Mercury. The radioscience observations acquired during the orbital phase of MESSENGER drastically improved our knowledge of the orbit of Mercury. An accurate MESSENGER orbit is obtained by fitting one-and-half years of tracking data using GINS orbit determination software. The systematic error in the Earth-Mercury geometric positions, also called range bias, obtained from GINS are then used to fit the INPOP dynamical modeling of the planet motions. An improved ephemeris of the planets is then obtained, INPOP13a, and used to perform general relativity tests of the parametrized post-Newtonian (PPN) formalism. -
Go, No-Go for Apollo Based on Orbital Life Time
https://ntrs.nasa.gov/search.jsp?R=19660014325 2020-03-24T02:45:56+00:00Z I L GO, NO-GO FOR APOLLO BASED ON ORBITAL LIFE TIME I hi 01 GPO PRICE $ Q N66(ACCESSION -2361 NUMBER1 4 ITHRU) Pf - CFSTI PRICE(S) $ > /7 (CODE) IPAGES) 'I 2 < -55494 (CATEGORY) f' (NASA CR OR rwx OR AD NUMBER) Hard copy (HC) /# d-a I Microfiche (M F) I By ff653 Julv65 F. 0. VONBUN NOVEMBER 1965 t GODDARD SPACE FLIGHT CENTER GREENBELT, MARYLAND ABSTRACT A simple expression for the go, no-go criterion is derived in analytical form. This provides a better understanding of the prob- lems involved which is lacking when large computer programs are used. A comparison between "slide rule" results and computer re- sults is indicated on Figure 2. An example is given using a 185 km near circular parking or- bit. It is shown that a 3a speed error of 6 m/s and a 3c~flight path angle error of 4.5 mrad result in a 3c~perigee error of 30 km which is tolerable when a 5 day orbital life time of the Apollo (SIVB + Service + Command Module) is required. iii CONTENTS Page INTRODUCTION ...................................... 1 I. VARLATIONAL EQUATION FOR THE PERIGEE HEIGHT h,. 1 11. THE ERROR IN THE ORBITAL PERIGEE HEIGHT ah,. 5 III. CRITERION FOR GO, NO-GO IN SIMPLE FORM . 5 REFERENCES ....................................... 10 LIST OF ILLUSTRATIONS Figure Page 1 Orbital Insertion Geometry . 2 2 Perigee Error for Apollo Parking Orbits (e 0, h, = 185 km) . 6 3 Height of the Apollo Parking Orbit After Insertion . -
PHY140Y 3 Properties of Gravitational Orbits
PHY140Y 3 Properties of Gravitational Orbits 3.1 Overview • Orbits and Total Energy • Elliptical, Parabolic and Hyperbolic Orbits 3.2 Characterizing Orbits With Energy Two point particles attracting each other via gravity will cause them to move, as dictated by Newton’s laws of motion. If they start from rest, this motion will simply be along the line separating the two points. It is a one-dimensional problem, and easily solved. The two objects collide. If the two objects are given some relative motion to start with, then it is easy to convince yourself that a collision is no longer possible (so long as these particles are point-like). In our discussion of orbits, we will only consider the special case where one object of mass M is much heavier than the smaller object of mass m. In this case, although the larger object is accelerated toward the smaller one, we will ignore that acceleration and assume that the heavier object is fixed in space. There are three general categories of motion, when there is some relative motion, which we can characterize by the total energy of the object, i.e., E ≡ K + U (1) 1 GMm = mv2 − , (2) 2 r2 where K and U are the kinetic and gravitational energy, respectively. 3.3 Energy < 0 In this case, since the total energy is negative, it is not possible for the object to get too far from the mass M, since E = K + U<0 (3) 1 GMm ⇒ mv2 = + E (4) 2 r GM ⇒ r = (5) 1 2 − E 2 v m GM ⇒ r< , (6) −E since r will take on its maximum value when the denominator is minimized (or when v is the smallest value possible, ie. -
Spacex Rocket Data Satisfies Elementary Hohmann Transfer Formula E-Mail: [email protected] and [email protected]
IOP Physics Education Phys. Educ. 55 P A P ER Phys. Educ. 55 (2020) 025011 (9pp) iopscience.org/ped 2020 SpaceX rocket data satisfies © 2020 IOP Publishing Ltd elementary Hohmann transfer PHEDA7 formula 025011 Michael J Ruiz and James Perkins M J Ruiz and J Perkins Department of Physics and Astronomy, University of North Carolina at Asheville, Asheville, NC 28804, United States of America SpaceX rocket data satisfies elementary Hohmann transfer formula E-mail: [email protected] and [email protected] Printed in the UK Abstract The private company SpaceX regularly launches satellites into geostationary PED orbits. SpaceX posts videos of these flights with telemetry data displaying the time from launch, altitude, and rocket speed in real time. In this paper 10.1088/1361-6552/ab5f4c this telemetry information is used to determine the velocity boost of the rocket as it leaves its circular parking orbit around the Earth to enter a Hohmann transfer orbit, an elliptical orbit on which the spacecraft reaches 1361-6552 a high altitude. A simple derivation is given for the Hohmann transfer velocity boost that introductory students can derive on their own with a little teacher guidance. They can then use the SpaceX telemetry data to verify the Published theoretical results, finding the discrepancy between observation and theory to be 3% or less. The students will love the rocket videos as the launches and 3 transfer burns are very exciting to watch. 2 Introduction Complex 39A at the NASA Kennedy Space Center in Cape Canaveral, Florida. This launch SpaceX is a company that ‘designs, manufactures and launches advanced rockets and spacecraft. -
Interplanetary Trajectory Design Using Dynamical Systems Theory THESIS REPORT by Linda Van Der Ham
Interplanetary Trajectory Design using Dynamical Systems Theory THESIS REPORT by Linda van der Ham 8 February 2012 The image on the front is an artist impression of the Interplanetary Super- highway [NASA, 2002]. Preface This MSc. thesis forms the last part of the curriculum of Aerospace Engineering at Delft University of Technology in the Netherlands. It covers subjects from the MSc. track Space Exploration, focusing on astrodynamics and space missions, as well as new theory as studied in the literature study performed previously. Dynamical Systems Theory was one of those new subjects, I had never heard of before. The existence of a so-called Interplanetary Transport Network intrigued me and its use for space exploration would mean a total new way of transfer. During the literature study I did not find any practical application of the the- ory to interplanetary flight, while it was stated as a possibility. This made me wonder whether it was even an option, a question that could not be answered until the end of my thesis work. I would like to thank my supervisor Ron Noomen, for his help and enthusi- asm during this period; prof. Wakker for his inspirational lectures about the wonders of astrodynamics; the Tudat group, making research easier for a lot of students in the future. My parents for their unlimited support in every way. And Wouter, for being there. Thank you all and enjoy! i ii Abstract In this thesis, manifolds in the coupled planar circular restricted three-body problem (CR3BP) as means of interplanetary transfer are examined. The equa- tions of motion or differential equations of the CR3BP are studied according to Dynamical Systems Theory (DST). -
Hyperbolic'type Orbits in the Schwarzschild Metric
Hyperbolic-Type Orbits in the Schwarzschild Metric F.T. Hioe* and David Kuebel Department of Physics, St. John Fisher College, Rochester, NY 14618 and Department of Physics & Astronomy, University of Rochester, Rochester, NY 14627, USA August 11, 2010 Abstract Exact analytic expressions for various characteristics of the hyperbolic- type orbits of a particle in the Schwarzschild geometry are presented. A useful simple approximation formula is given for the case when the deviation from the Newtonian hyperbolic path is very small. PACS numbers: 04.20.Jb, 02.90.+p 1 Introduction In a recent paper [1], we presented explicit analytic expressions for all possible trajectories of a particle with a non-zero mass and for light in the Schwarzschild metric. They include bound, unbound and terminating orbits. We showed that all possible trajectories of a non-zero mass particle can be conveniently characterized and placed on a "map" in a dimensionless parameter space (e; s), where we have called e the energy parameter and s the …eld parameter. One region in our parameter space (e; s) for which we did not present extensive data and analysis is the region for e > 1. The case for e > 1 includes unbound orbits that we call hyperbolic-type as well as terminating orbits. In this paper, we consider the hyperbolic-type orbits in greater detail. In Section II, we …rst recall the explicit analytic solution for the elliptic- (0 e < 1), parabolic- (e = 1), and hyperbolic-type (e > 1) orbits in what we called Region I (0 s s1), where the upper boundary of Region I characterized by s1 varies from s1 = 0:272166 for e = 0 to s1 = 0:250000 for e = 1 to s1 0 as e . -
Trajectory Design Tools for Libration and Cis-Lunar Environments
Trajectory Design Tools for Libration and Cis-Lunar Environments David C. Folta, Cassandra M. Webster, Natasha Bosanac, Andrew Cox, Davide Guzzetti, and Kathleen C. Howell National Aeronautics and Space Administration/ Goddard Space Flight Center, Greenbelt, MD, 20771 [email protected], [email protected] School of Aeronautics and Astronautics, Purdue University, West Lafayette, IN 47907 {nbosanac,cox50,dguzzett,howell}@purdue.edu ABSTRACT science orbit. WFIRST trajectory design is based on an optimal direct-transfer trajectory to a specific Sun- Innovative trajectory design tools are required to Earth L2 quasi-halo orbit. support challenging multi-body regimes with complex dynamics, uncertain perturbations, and the integration Trajectory design in support of lunar and libration of propulsion influences. Two distinctive tools, point missions is becoming more challenging as more Adaptive Trajectory Design and the General Mission complex mission designs are envisioned. To meet Analysis Tool have been developed and certified to these greater challenges, trajectory design software provide the astrodynamics community with the ability must be developed or enhanced to incorporate to design multi-body trajectories. In this paper we improved understanding of the Sun-Earth/Moon discuss the multi-body design process and the dynamical solution space and to encompass new capabilities of both tools. Demonstrable applications to optimal methods. Thus the support community needs confirmed missions, the Lunar IceCube Cubesat lunar to improve the efficiency and expand the capabilities mission and the Wide-Field Infrared Survey Telescope of current trajectory design approaches. For example, (WFIRST) Sun-Earth L2 mission, are presented. invariant manifolds, derived from dynamical systems theory, have been applied to the trajectory design of 1.