Behavioural, Convergent and Ontogenetic Evidence for Adaptive Behavioural Manipulation in Mermithid Infected Hosts
Total Page:16
File Type:pdf, Size:1020Kb
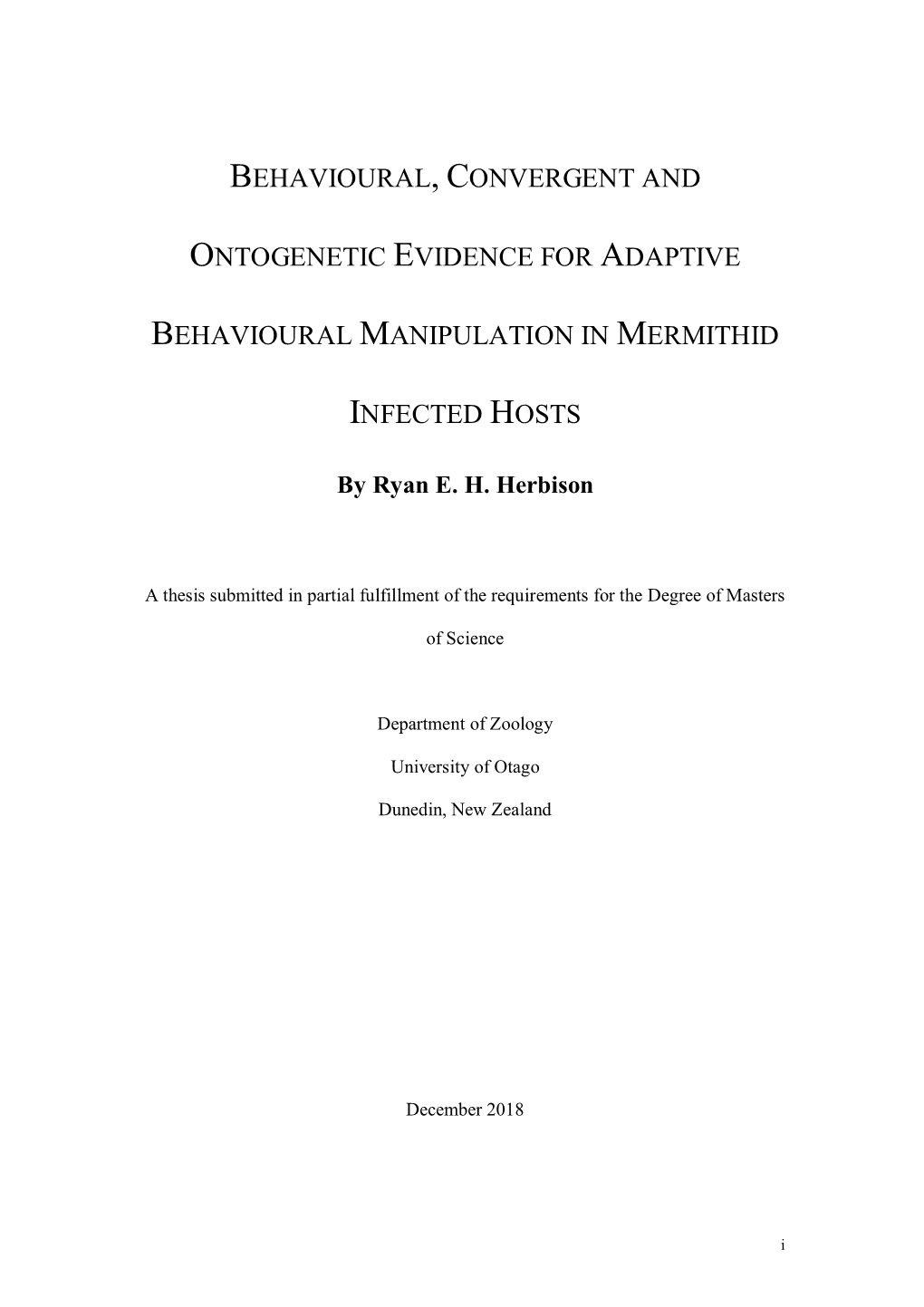
Load more
Recommended publications
-
Water-Seeking Behavior in Worm-Infected Crickets and Reversibility of Parasitic Manipulation
View metadata, citation and similar papers at core.ac.uk brought to you by CORE provided by HAL Clermont Université Water-seeking behavior in worm-infected crickets and reversibility of parasitic manipulation. Fleur Ponton, Fernando Ot´alora-Luna,Thierry Lef`evre,Patrick M Guerin, Camille Lebarbenchon, David Duneau, David Georges Biron, Fr´ed´ericThomas To cite this version: Fleur Ponton, Fernando Ot´alora-Luna,Thierry Lef`evre,Patrick M Guerin, Camille Lebarben- chon, et al.. Water-seeking behavior in worm-infected crickets and reversibility of parasitic manipulation.. Behavioral Ecology, Oxford University Press (OUP), 2011, 22 (2), pp.392-400. <10.1093/beheco/arq215>. <hal-00814753> HAL Id: hal-00814753 https://hal.archives-ouvertes.fr/hal-00814753 Submitted on 17 Apr 2013 HAL is a multi-disciplinary open access L'archive ouverte pluridisciplinaire HAL, est archive for the deposit and dissemination of sci- destin´eeau d´ep^otet `ala diffusion de documents entific research documents, whether they are pub- scientifiques de niveau recherche, publi´esou non, lished or not. The documents may come from ´emanant des ´etablissements d'enseignement et de teaching and research institutions in France or recherche fran¸caisou ´etrangers,des laboratoires abroad, or from public or private research centers. publics ou priv´es. Behavioral Ecology doi:10.1093/beheco/arq215 Advance Access publication 8 February 2011 Original Article Water-seeking behavior in worm-infected crickets and reversibility of parasitic manipulation Fleur Ponton,a Fernando Ota´lora-Luna,b Thierry Lefe`vre,a Patrick M. Guerin,b Camille Lebarbenchon,a David Duneau,a David G. Biron,a and Fre´de´ric Thomasa aGEMI/UMR CNRS-IRD 2724, Equipe: ‘‘Evolution des Syste`mes Symbiotiques’’, IRD, 911 Avenue Agropolis, B.P. -
THE QUARTERLY REVIEW of BIOLOGY
VOL. 43, NO. I March, 1968 THE QUARTERLY REVIEW of BIOLOGY LIFE CYCLE ORIGINS, SPECIATION, AND RELATED PHENOMENA IN CRICKETS BY RICHARD D. ALEXANDER Museum of Zoology and Departmentof Zoology The Universityof Michigan,Ann Arbor ABSTRACT Seven general kinds of life cycles are known among crickets; they differ chieff,y in overwintering (diapause) stage and number of generations per season, or diapauses per generation. Some species with broad north-south ranges vary in these respects, spanning wholly or in part certain of the gaps between cycles and suggesting how some of the differences originated. Species with a particular cycle have predictable responses to photoperiod and temperature regimes that affect behavior, development time, wing length, bod)• size, and other characteristics. Some polymorphic tendencies also correlate with habitat permanence, and some are influenced by population density. Genera and subfamilies with several kinds of life cycles usually have proportionately more species in temperate regions than those with but one or two cycles, although numbers of species in all widely distributed groups diminish toward the higher lati tudes. The tendency of various field cricket species to become double-cycled at certain latitudes appears to have resulted in speciation without geographic isolation in at least one case. Intermediate steps in this allochronic speciation process are illustrated by North American and Japanese species; the possibility that this process has also occurred in other kinds of temperate insects is discussed. INTRODUCTION the Gryllidae at least to the Jurassic Period (Zeuner, 1939), and many of the larger sub RICKETS are insects of the Family families and genera have spread across two Gryllidae in the Order Orthoptera, or more continents. -
Invasion of the Body Snatchers: the Diversity and Evolution of Manipulative Strategies in Host–Parasite Interactions
CHAPTER 3 Invasion of the Body Snatchers: The Diversity and Evolution of Manipulative Strategies in Host–Parasite Interactions † Thierry Lefe`vre,* Shelley A. Adamo, ‡ David G. Biron, Dorothe´e Misse´,* § k } David Hughes, , ,1 and Fre´de´ric Thomas*, ,1 Contents 3.1. Introduction 46 3.2. How Parasites Alter Host Behaviour 49 3.2.1. Parasitic effects on host neural function 49 3.2.2. Proteomics and proximate mechanisms 56 3.3. A Co-Evolutionary Perspective 66 3.3.1. Exploiting host-compensatory responses 66 3.2.2. Facultative virulence 72 3.4. The (River) Blind Watchmaker 75 3.5. Concluding Remarks 76 References 76 Abstract Parasite-induced alteration of host behaviour is a widespread trans- mission strategy among pathogens. Understanding how it works is an exciting challenge from both a mechanistic and an evolutionary perspective. In this review, we use key examples to examine the * Ge´ne´tique et Evolution des Maladies Infectieuses, UMR CNRS/IRD 2724, Montpellier, France { Department of Psychology, Dalhousie University, Halifax, Canada { PIAF, UMR 547 INRA/Universite´ Blaise-Pascal, Clermont-Ferrand, France § Department of Organismal Biology, University of Harvard, Cambridge, MA, USA } Institut de recherche en biologie ve´ge´tale, De´partement de sciences biologiques Universite´ de Montre´al, Montre´al, Que´bec, Canada k School of Biosciences, University of Exeter, Exeter, UK 1 Both authors contributed equally Advances in Parasitology, Volume 68 # 2009 Elsevier Ltd. ISSN 0065-308X, DOI: 10.1016/S0065-308X(08)00603-9 All rights reserved. 45 46 Thierry Lefe`vre et al. proximate mechanisms by which parasites are known to control the behaviour of their hosts. -
ED45E Rare and Scarce Species Hierarchy.Pdf
104 Species 55 Mollusc 8 Mollusc 334 Species 181 Mollusc 28 Mollusc 44 Species 23 Vascular Plant 14 Flowering Plant 45 Species 23 Vascular Plant 14 Flowering Plant 269 Species 149 Vascular Plant 84 Flowering Plant 13 Species 7 Mollusc 1 Mollusc 42 Species 21 Mollusc 2 Mollusc 43 Species 22 Mollusc 3 Mollusc 59 Species 30 Mollusc 4 Mollusc 59 Species 31 Mollusc 5 Mollusc 68 Species 36 Mollusc 6 Mollusc 81 Species 43 Mollusc 7 Mollusc 105 Species 56 Mollusc 9 Mollusc 117 Species 63 Mollusc 10 Mollusc 118 Species 64 Mollusc 11 Mollusc 119 Species 65 Mollusc 12 Mollusc 124 Species 68 Mollusc 13 Mollusc 125 Species 69 Mollusc 14 Mollusc 145 Species 81 Mollusc 15 Mollusc 150 Species 84 Mollusc 16 Mollusc 151 Species 85 Mollusc 17 Mollusc 152 Species 86 Mollusc 18 Mollusc 158 Species 90 Mollusc 19 Mollusc 184 Species 105 Mollusc 20 Mollusc 185 Species 106 Mollusc 21 Mollusc 186 Species 107 Mollusc 22 Mollusc 191 Species 110 Mollusc 23 Mollusc 245 Species 136 Mollusc 24 Mollusc 267 Species 148 Mollusc 25 Mollusc 270 Species 150 Mollusc 26 Mollusc 333 Species 180 Mollusc 27 Mollusc 347 Species 189 Mollusc 29 Mollusc 349 Species 191 Mollusc 30 Mollusc 365 Species 196 Mollusc 31 Mollusc 376 Species 203 Mollusc 32 Mollusc 377 Species 204 Mollusc 33 Mollusc 378 Species 205 Mollusc 34 Mollusc 379 Species 206 Mollusc 35 Mollusc 404 Species 221 Mollusc 36 Mollusc 414 Species 228 Mollusc 37 Mollusc 415 Species 229 Mollusc 38 Mollusc 416 Species 230 Mollusc 39 Mollusc 417 Species 231 Mollusc 40 Mollusc 418 Species 232 Mollusc 41 Mollusc 419 Species 233 -
Top 10 Real-Life Body Snatchers
Top 10 Real-Life Body Snatchers Parasites and zombies are not science fiction; they infest rats, crickets, ants, moths and other creatures, sucking the life out of them By Megan Gambino, Smithsonian.com October 24, 2011 To ensure their own survival, parasites alter the appearance and behavior of their hosts in the creepiest ways. For instance, rats carrying the parasitic protozoan Toxoplasma gondii, which reproduces inside the gut of a cat, no longer fear the smell of cat urine. In fact, they are sexually attracted to the scent, according to a recent study. This way, infected rats walk right into the grips of a feline. Here are ten other parasites whose sophisticated manipulations of animals are more horrifying than fiction. 1. Paragordius tricuspidatus Exactly how a hairworm parasitizes a cricket is unknown. Scientists suspect that the insect ingests either an infected mosquito or water containing hairworm larvae. But once inside, the hairworm grows three to four times as long as the arthropod, filling all parts of its body except the head and legs. What happens next is even more bizarre. The parasite, Paragordius tricuspidatus, produces proteins that hijack the cricket’s central nervous system, making it attracted to areas brighter than its shaded forest home. The cricket, Nemobius sylvestris, heads then to an exposed pond or river and dives in, at which point the hairworm emerges from its host’s rear end. In an aquatic environment, the worm can find a mate and reproduce. For some crickets, it’s a suicide leap. But others lucky enough not to have drowned have lived for several months after the parasite removes itself. -
Orthoptera, Gryllidae, Nemobiinae) Discovered in Colluvial, Stony Debris in the Iberian Peninsula: a Biological, Phenological and Biometric Study
Zootaxa 3691 (2): 201–219 ISSN 1175-5326 (print edition) www.mapress.com/zootaxa/ Article ZOOTAXA Copyright © 2013 Magnolia Press ISSN 1175-5334 (online edition) http://dx.doi.org/10.11646/zootaxa.3691.2.1 http://zoobank.org/urn:lsid:zoobank.org:pub:860039CB-3547-4088-B4CE-0DD240559ACA A new mute species of the genus Nemobius Serville (Orthoptera, Gryllidae, Nemobiinae) discovered in colluvial, stony debris in the Iberian Peninsula: A biological, phenological and biometric study PABLO BARRANCO1,3, JOSÉ D. GILGADO2 & VICENTE M. ORTUÑO2 1Dpto. Biología y Geología. Cite II-B. Universidad de Almería. E-04120 – Almería. Spain 2Dpto. de Ciencias de la Vida. Facultad de Biología, Ciencias Ambientales y Química. Universidad de Alcalá, E-28871 – Alcalá de Henares. Madrid. Spain 3Corresponding author. E-mail: [email protected] Abstract Sampling of a Mesovoid Shallow Substratum (MSS) of a scree in the Guadarrama mountains (Madrid, Spain) revealed a population of crickets of the genus Nemobius Serville. A detailed morphological study revealed that the cricket was a new species, Nemobius interstitialis sp. nov., which is principally characterized by the absence of a tympanum in the outer margin of the foreleg tibiae and a peculiar design of venation of the forewing of the male. Sampling of this environment over 1 year using surface and MSS pitfall traps, set at a depth of one meter, allowed study of population dynamics. A pop- ulation maximum is attained in August. Abiotic (temperature and humidity) and biotic (accompanying fauna) data are giv- en to contextualize the habitat of this new species. Key words: Nemobius interstitialis sp. nov., biology, Mesovoid Shallow Substratum (MSS), Guadarrama mountains, Ibe- rian Peninsula. -
JESBC 2007 91-92 Cannings.Pub
J. ENTOMOL. SOC. BRIT. COLUMBIA 104, DECEMBER 2007 91 SCIENTIFIC NOTE Meconema thalassinum (Orthoptera: Tettigoniidae), a foreign katydid established in British Columbia ROBERT A. CANNINGS1, JAMES W. MISKELLY2, CATRIEN A.H. SCHIFFER3, KAR LUN ALAN LAU4 and KAREN M. NEEDHAM4 Meconema thalassinum (De Geer), a from the Lower Mainland region of south- katydid in the tettigoniid subfamily Me- western British Columbia (from the Greater conematinae, is a European native estab- Vancouver area east to Maple Ridge and lished in northeastern North America since Langley) between 1991 and 2007 1957 (Capinera et al. 2004). In the pub- (Collections deposited in RBCM – Royal lished literature it is known as far west as British Columbia Museum, Victoria, BC; Michigan and extreme southwestern On- SFU – Simon Fraser University collection, tario (Marshall et al. 2004). Burnaby, BC; UBC – Spencer Entomologi- Rather than stridulating by rubbing the cal Museum, University of BC, Vancouver, forewings together, as most Ensifera do, BC). Most specimens (25 of 31) were col- males call at night by tapping their hind lected by entomology students for class tarsi on leaf surfaces or other substrates, projects at Simon Fraser University and the and thus M. thalassinum is known in North University of British Columbia. The earliest America as the Drumming Katydid. The records are from Surrey (16 September drumming is quiet, but sometimes may be 1991, J. Mayer (SFU)) and Haney (22 Sep- heard 3 to 4 m away (Capinera et al. 2004). tember 1991, S. Chaabra (SFU)). The species is pale green with a dorsal yel- David Holden (pers. comm.), trapping low stripe on the head and prothorax. -
Entomologist's Gazette 61
2010, Entomologist’s Gazette 61: 53–64 Hedgerow species richness influences the presence of Orthoptera and Dermaptera along green lanes in Essex, U.K. Tim Gardiner 2 Beech Road, Rivenhall,Witham, Essex CM8 3PF,U.K. [email protected] Synopsis a small-scale beating survey of the Orthoptera (bush-crickets) and dermaptera (earwigs) of green lane hedgerows was undertaken in essex. Hedgerow dating (Hooper’s rule) was used to ascertain whether insects were related to woody plant species richness. Orthopteroid abundance and species richness was significantly correlated with the number of woody plants in the hedgerows. Byways had particularly species-rich, ancient hedgerows (often over 500 years in age) favourable for bush-crickets such as Meconema thalassinum. Sunlit patches of Blackthorn Prunus spinosa and Bramble Rubus fruticosus hedgerow were especially important for orthopteroids in this survey. Keywords: earwig, bush-cricket, footpath, bridleway, byway, highways, Hooper’s rule, microclimate. Introduction Green lanes (double-hedged tracks) are important for insects, particularly butterflies, in areas of intensive agriculture (dover & Sparks, 2001). Hedgerows can also be valuable corridors for the dispersal of sedentary insects such as Lampyris noctiluca (Linnaeus) (Gardiner, 2008b), particularly along green lanes in epping Forest where the beetle has declined in the last 50 years (Gardiner, 2007c). Hedgerows provide sheltered conditions where reduced wind speed can create a warm microclimate suitable for insects (dover, Sparks & Greatorex-davies, 1997; Gardiner & dover, 2008). Green lanes often have public rights of way (PrOW) running down them, the most common are public footpaths (pedestrian use only), bridleways (use by pedestrians, cyclists and horse riders) and byways (open to all traffic: use by pedestrians, cyclists, horse riders, horse drawn and motorised vehicles). -
Diversity and Distribution of Orthoptera Communities of Two Adjacent Mountains in Northern Part of the Carpathians
Travaux du Muséum National d’Histoire Naturelle “Grigore Antipa” 62 (2): 191–211 (2019) doi: 10.3897/travaux.62.e48604 RESEARCH ARTICLE Diversity and distribution of Orthoptera communities of two adjacent mountains in northern part of the Carpathians Anton Krištín1, Benjamín Jarčuška1, Peter Kaňuch1 1 Institute of Forest Ecology SAS, Ľ. Štúra 2, Zvolen, SK-96053, Slovakia Corresponding author: Anton Krištín ([email protected]) Received 19 November 2019 | Accepted 24 December 2019 | Published 31 December 2019 Citation: Krištín A, Jarčuška B, Kaňuch P (2019) Diversity and distribution of Orthoptera communities of two adjacent mountains in northern part of the Carpathians. Travaux du Muséum National d’Histoire Naturelle “Grigore Antipa” 62(2): 191–211. https://doi.org/10.3897/travaux.62.e48604 Abstract During 2013–2017, assemblages of bush-crickets and grasshoppers were surveyed in two neighbour- ing flysch mountains – Čergov Mts (48 sites) and Levočské vrchy Mts (62 sites) – in northern part of Western Carpathians. Species were sampled mostly at grasslands and forest edges along elevational gradient between 370 and 1220 m a.s.l. Within the entire area (ca 930 km2) we documented 54 species, representing 38% of Carpathian Orthoptera species richness. We found the same species number (45) in both mountain ranges with nine unique species in each of them. No difference in mean species rich- ness per site was found between the mountain ranges (mean ± SD = 12.5 ± 3.9). Elevation explained 2.9% of variation in site species richness. Elevation and mountain range identity explained 7.3% of assemblages composition. We found new latitudinal as well as longitudinal limits in the distribu- tion for several species. -
The Song Relationships of Four Species of Ground Crickets (Orthoptera: Gryllidae: Nemobius)1
THE SONG RELATIONSHIPS OF FOUR SPECIES OF GROUND CRICKETS (ORTHOPTERA: GRYLLIDAE: NEMOBIUS)1 RICHARD D. ALEXANDER Department of Zoology and Entomology, The Ohio State University, Columbus 10 INTRODUCTION Several accounts have been written of the mating behavior of different species of ground crickets (Fulton, 1931; Richards, 1952; Gabbutt, 1954), and many excellent descriptions of their songs, based on auditory impressions, have appeared (Fulton, 1930, 1931, 1932; Cantrall, 1943; and many others). A few song analyses have been made with mechanical or electronic devices (Fulton, 1933; Pielemeier, 1946, 1946a; Pierce, 1948). Detailed comparative studies of song relationships in the singing Orthoptera and Cicadidae are almost non-existent, though it is increasingly apparent that satisfactory interpretations of the taxonomic and distri- butional relationships of these species will depend on such studies. The present investigation is based on laboratory and field observations, and on tape recordings of songs analyzed by means of a Vibralyzer. The species dis- cussed include Nemobius carolinus carolinus Scudder, N. confusus Blatchley, and N. melodius Thomas and Alexander, the only eastern representatives of the sub- genus Eunemobius Hebard. A fourth species, N. maculatus Blatchley, belonging in the subgenus Allonemobius Hebard, is also considered here because of certain distributional and song relationships with the above species. The recordings used for song analysis are deposited in the Library of Animal Sounds, Department of Zoology and Entomology, The Ohio State University. They were made with a Magnemite, Model 610-E (in the field) and a Magnecorder, Model PT6A (in the laboratory) at a tape speed of fifteen inches per second. American Microphone Company Microphones, Models D-33 and D-33A, were used, both in the field and in the laboratory. -
New Canadian and Ontario Orthopteroid Records, and an Updated Checklist of the Orthoptera of Ontario
Checklist of Ontario Orthoptera (cont.) JESO Volume 145, 2014 NEW CANADIAN AND ONTARIO ORTHOPTEROID RECORDS, AND AN UPDATED CHECKLIST OF THE ORTHOPTERA OF ONTARIO S. M. PAIERO1* AND S. A. MARSHALL1 1School of Environmental Sciences, University of Guelph, Guelph, Ontario, Canada N1G 2W1 email, [email protected] Abstract J. ent. Soc. Ont. 145: 61–76 The following seven orthopteroid taxa are recorded from Canada for the first time: Anaxipha species 1, Cyrtoxipha gundlachi Saussure, Chloroscirtus forcipatus (Brunner von Wattenwyl), Neoconocephalus exiliscanorus (Davis), Camptonotus carolinensis (Gerstaeker), Scapteriscus borellii Linnaeus, and Melanoplus punctulatus griseus (Thomas). One further species, Neoconocephalus retusus (Scudder) is recorded from Ontario for the first time. An updated checklist of the orthopteroids of Ontario is provided, along with notes on changes in nomenclature. Published December 2014 Introduction Vickery and Kevan (1985) and Vickery and Scudder (1987) reviewed and listed the orthopteroid species known from Canada and Alaska, including 141 species from Ontario. A further 15 species have been recorded from Ontario since then (Skevington et al. 2001, Marshall et al. 2004, Paiero et al. 2010) and we here add another eight species or subspecies, of which seven are also new Canadian records. Notes on several significant provincial range extensions also are given, including two species originally recorded from Ontario on bugguide.net. Voucher specimens examined here are deposited in the University of Guelph Insect Collection (DEBU), unless otherwise noted. New Canadian records Anaxipha species 1 (Figs 1, 2) (Gryllidae: Trigidoniinae) This species, similar in appearance to the Florida endemic Anaxipha calusa * Author to whom all correspondence should be addressed. -
Tettigoniidae: Saginae), an Old World Katydid, New to Michigan
The Great Lakes Entomologist Volume 5 Number 3 -- Fall 1972 Number 3 -- Fall 1972 Article 11 August 1972 Saga Pedo (Pallas) (Tettigoniidae: Saginae), an Old World Katydid, New to Michigan Irving J. Cantrall University of Michigan Follow this and additional works at: https://scholar.valpo.edu/tgle Part of the Entomology Commons Recommended Citation Cantrall, Irving J. 1972. "Saga Pedo (Pallas) (Tettigoniidae: Saginae), an Old World Katydid, New to Michigan," The Great Lakes Entomologist, vol 5 (3) Available at: https://scholar.valpo.edu/tgle/vol5/iss3/11 This Peer-Review Article is brought to you for free and open access by the Department of Biology at ValpoScholar. It has been accepted for inclusion in The Great Lakes Entomologist by an authorized administrator of ValpoScholar. For more information, please contact a ValpoScholar staff member at [email protected]. Cantrall: <i>Saga Pedo</i> (Pallas) (Tettigoniidae: Saginae), an Old World 1972 THE GREAT LAKES ENTOMOLOGIST 103 SAGA PEDO (PALLAS) (TETTIGONIIDAE: SAGINAE), AN OLD WORLD KATYDID, NEW TO MICHIGAN Irving J. Cantrall Museum of Zoology, University of Michigan, Ann Arbor, Michigan 48104 At least four species of Old World Tettigoniidae are known to have been introduced into, and to have become established in the United States. One of these, Phaneroptera quadripunctata Brunner was first taken at Niles, California in 1932 and was reported by Strohecker (1952). The other three have been taken during the past two decades. Strohecker (1955) recorded Platycleis tessellata (Charpentier) from a specimen captured at Placemille, California in 1951, Urquart and Beaudry (1953) recorded Metrioptera roeseli (Hagenbach) as occurring at Ville Saint-Laurent and at Montrdal, Qudbec, Canada in 1952, and Gurney (1960) stated that the first specimens of Meconema thalassinum (De Geer) were taken at Little Neck, Long Island in 1959.