Trophic Interactions of Megafauna in the Mariana and Kermadec Trenches Inferred from Stable Isotope Analysis
Total Page:16
File Type:pdf, Size:1020Kb
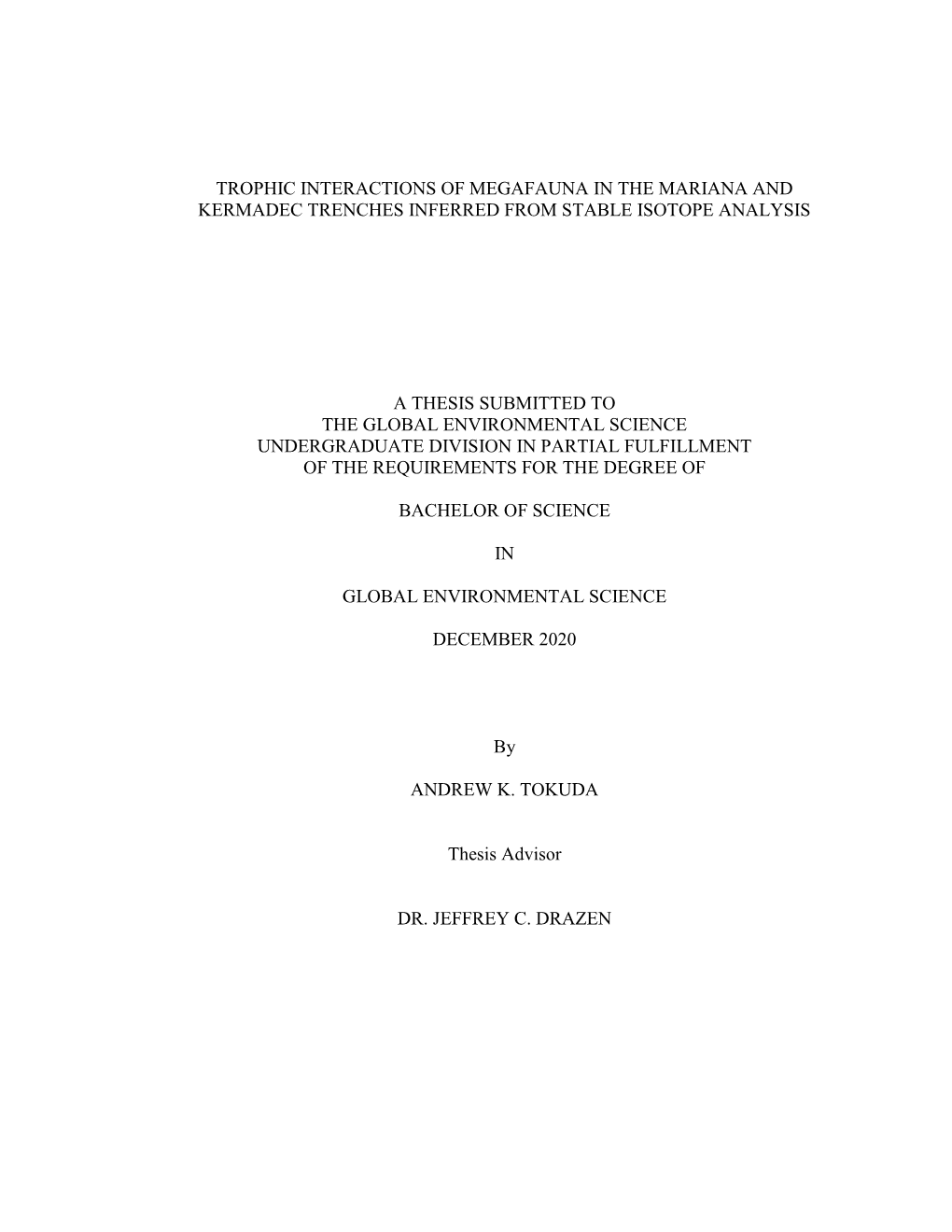
Load more
Recommended publications
-
Stagnant Slabs in the Upper and Lower Mantle Transition Region
STAGNANT SLABS IN THE UPPER AND LOWER MANTLE TRANSITION REGION Yoshio Fukao Sri Widiyantoro MasayukiObayashi • EarthquakeResearch Institute Departmentof Geophysics Seismologyand Volcanology Universityof Tokyo and Meteorology ResearchDepartment Tokyo,Japan BandungInstitute of Technology MeteorologicalResearch Bandung,Indonesia Institute Tsukuba,Japan Abstract. We made a region-by-regionexamination of with present slab subduction appears to be blocked subductedslab images along the circum-Pacificfor some stronglyto turn into predominantlyhorizontal flow in of the recentglobal mantle tomographicmodels, specif- the transitionregion. Recent globaltomographic models ically for two high-resolutionP velocitymodels and two show also a group of lithosphericslabs deeply sinking long-wavelengthS velocity models. We extractedthe throughthe lower mantle, typicallythe presumedFaral- slab imagesthat are most consistentamong different lon slab beneath North and Central America and the models. We found that subducted slabs tend to be sub- presumedIndian (Tethys) slab beneathHimalaya and horizontally deflected or flattened in the upper and the Bay of Bengal. These remnant slabs are not con- lower mantle transitionregion, the depth range of which nectedto the surfaceplates or to the presentlysubduct- correspondsroughly to the Bullen transition region ing slabs and appear to sink independentlyfrom the (400-1000 km). The deflectedor flattenedslabs reside latter. The presenceof thesedeeply sinking slabs implies at different depths,either above or acrossthe 660-km that the pre-Eocene subductionoccurred in much the discontinuityas in Chile Andes, Aleutian, Southern sameway asin the presentday to accumulateslab bodies Kurile, Japan,and Izu-Bonin; slightlybelow the discon- in the transitionregion and that the consequentunstable tinuity as in Northern Kurile, Mariana, and Philippine; downflow occurred extensivelythrough the transition or well below it as in Peru Andes, Java, and Tonga- region in the Eocene epoch to detach many of the Kermadec. -
NOAA Technical Report NOS NGS 74 (Rotation
NOAA Technical Report NOS NGS 74 A GPS Based Estimate of the Rotation of the Mariana Plate in both ITRF2008 and ITRF2014 Dru Smith Silver Spring, MD August 2020 National Oceanic and Atmospheric Administration National Geodetic Survey Versions Date Changes August 11, 2020 Original Release 2 Publication Notes Part 1 of this report was originally submitted to the Journal of Geodesy in 2018. I would like to personally thank the three reviewers who provided extensive and useful feedback (Donald Argus, Corné Kreemer and a third anonymous reviewer.) The original paper provided a rotation of the Mariana plate in ITRF2008, and is presented in Part 1 of this report. During the intervening years the geodetic world moved on from ITRF2008 to ITRF2014. Further, new surveys in Guam and CNMI added information not in the original paper. As such, a complete re-processing of the data allowed the original ITRF2008 rotation to be updated to ITRF2014, as well as to provide the opportunity to compare against, and possibly incorporate the new surveys. This information is presented in Part 2 of this report. The motivation behind these studies has been for NGS to have the ability to compute an accurate rotation of the Mariana plate within the ITRF, for use in defining the forthcoming MATRF2022 reference frame (NGS, 2017a). That relationship will likely be in the ITRF2020 frame, and since the lessons learned from both the ITRF2008 and ITRF2014 studies may inform the determination of the Mariana plate within ITRF2020, both are presented herein. 3 PART 1: Rotation of the Mariana Plate in ITRF2008 4 1 Introduction This paper represents the results of using GPS data collected by the National Oceanic and Atmospheric Administration’s (NOAA’s) National Geodetic Survey (NGS), to determine the rotational behavior of the Mariana Plate within an absolute frame (ITRF2008). -
Review of Tsunamigenic Sources of the Bay of Plenty Region, GNS Science Consultancy Report 2011/224
DISCLAIMER This report has been prepared by the Institute of Geological and Nuclear Sciences Limited (GNS Science) exclusively for and under contract to Bay of Plenty regional Council. Unless otherwise agreed in writing by GNS Science, GNS Science accepts no responsibility for any use of, or reliance on any contents of this Report by any person other than Bay of Plenty regional Council and shall not be liable to any person other than Bay of Plenty regional Council, on any ground, for any loss, damage or expense arising from such use or reliance. The data presented in this Report are available to GNS Science for other use from June 2012. BIBLIOGRAPHIC REFERENCE Prasetya, G. and Wang, X. 2011. Review of tsunamigenic sources of the Bay of Plenty region, GNS Science Consultancy Report 2011/224. 74 p. Project Number: 410W1369 Confidential 2011 CONTENTS EXECUTIVE SUMMARY ....................................................................................................... VII 1.0 INTRODUCTION .......................................................................................................... 1 2.0 OVERVIEW OF PREVIOUS STUDIES ........................................................................ 1 2.1 Joint Tsunami Research Project of EBOP and EW (Bell et al. 2004) ............................ 1 2.2 Tsunami Source Study (Goff et al. 2006) ....................................................................... 4 2.2.1 Mw 8.5 Scenarios.............................................................................................. 5 2.2.1.1 -
Visualization of the Geophysical Settings in the Philippine Sea Margins by Means of GMT and ISC Data
Central European Journal of Geography and Sustainable Development 2020, Volume 2, Issue 1, Pages: 5-15 ISSN 2668-4322, ISSN-L 2668-4322 https://doi.org/10.47246/CEJGSD.2020.2.1.1 Visualization of the geophysical settings in the Philippine Sea margins by means of GMT and ISC data Polina Lemenkova* Ocean University of China, College of Marine Geo-sciences, 238 Songling Rd, Laoshan, 266100, Qingdao, Shandong, China; [email protected] Received: 22 February 2020; Revised: 12 March 2020; Accepted: 20 March 2020; Published online: 25 March 2020 _________________________________________________________________________________________________________________________ Abstract: The presented research aimed to perform geophysical modelling (gravity and geoid) and to evaluate the spatio-temporal variation of the marine geological data (distribution and depth of earthquakes) using combination of the Generic Mapping Tools (GMT) and available sources from the International Seismological Centre (ISC-EHB) that produce data on earthquakes as part of seismic survey and regional research projects. The target study area is a Philippine Sea basin (PSB) with two focused marginal areas: Philippine Trench and Mariana Trench, two hadal trenches located in the places of the tectonic plates subduction. Marine free-air gravity anomaly in the PSP shows higher values (>80 mGal) of the gravity fields structure at the volcanic areas and Philippine archipelago. Current study presented comparative geophysical analysis, and mapping free-air gravity and geoid in the Philippine Sea basin area. As a result of this study, the average level of earthquakes located in the Philippine Trench and Mariana Trench areas were compared, and those located in the Philippine archipelago are determined to be in the souther-western part (area of west Mindanao, south-west Visayas islands), while Luzon Islands shown shallower located earthquakes. -
The Role of Body Size in Complex Food Webs: a Cold Case
Provided for non-commercial research and educational use only. Not for reproduction, distribution or commercial use. This chapter was originally published in the book Advances in Ecological Research, Vol. 45 published by Elsevier, and the attached copy is provided by Elsevier for the author's benefit and for the benefit of the author's institution, for non-commercial research and educational use including without limitation use in instruction at your institution, sending it to specific colleagues who know you, and providing a copy to your institution’s administrator. All other uses, reproduction and distribution, including without limitation commercial reprints, selling or licensing copies or access, or posting on open internet sites, your personal or institution’s website or repository, are prohibited. For exceptions, permission may be sought for such use through Elsevier's permissions site at: http://www.elsevier.com/locate/permissionusematerial From: Ute Jacob, Aaron Thierry, Ulrich Brose, Wolf E. Arntz, Sofia Berg, Thomas Brey, Ingo Fetzer, Tomas Jonsson, Katja Mintenbeck, Christian Möllmann, Owen Petchey, Jens O. Riede and Jennifer A. Dunne, The Role of Body Size in Complex Food Webs: A Cold Case. In Andrea Belgrano and Julia Reiss, editors: Advances in Ecological Research, Vol. 45, Amsterdam, The Netherlands, 2011, pp. 181-223. ISBN: 978-0-12-386475-8 © Copyright 2011 Elsevier Ltd. Academic press. Author's personal copy The Role of Body Size in Complex Food Webs: A Cold Case UTE JACOB,1,* AARON THIERRY,2,3 ULRICH BROSE,4 WOLF E. ARNTZ,5 SOFIA BERG,6 THOMAS BREY,5 INGO FETZER,7 TOMAS JONSSON,6 KATJA MINTENBECK,5 CHRISTIAN MO¨ LLMANN,1 OWEN L. -
Elpidia Soyoae, a New Species of Deep-Sea Holothurian (Echinodermata) from the Japan Trench Area
Species Diversity 25: 153–162 Published online 7 August 2020 DOI: 10.12782/specdiv.25.153 Elpidia soyoae, a New Species of Deep-sea Holothurian (Echinodermata) from the Japan Trench Area Akito Ogawa1,2,4, Takami Morita3 and Toshihiko Fujita1,2 1 Graduate School of Science, The University of Tokyo, 7-3-1 Hongo, Bunkyo-ku, Tokyo 113-0033, Japan E-mail: [email protected] 2 Department of Zoology, National Museum of Nature and Science, 4-1-1 Amakubo, Tsukuba, Ibaraki 305-0005, Japan 3 National Research Institute of Fisheries Science, Japan Fisheries Research and Education Agency, 2-12-4 Fukuura, Kanazawa-ku, Yokohama, Kanagawa 236-8648, Japan 4 Corresponding author (Received 23 October 2019; Accepted 28 May 2020) http://zoobank.org/00B865F7-1923-4F75-9075-14CB51A96782 A new species of holothurian, Elpidia soyoae sp. nov., is described from the Japan Trench area, at depths of 3570– 4145 m. It is distinguished from its congeners in having: four or five paired papillae and unpaired papillae present along entire dorsal radii (four to seven papillae on each radius), with wide separation between second and third paired papillae; maximum length of Elpidia-type ossicles in dorsal body wall exceeds 1000 µm; axis diameter of dorsal Elpidia-type ossicles less than 40 µm; tentacle Elpidia-type ossicles with arched axis and shortened, occasionally completely reduced arms and apophyses. Purple pigmentation spots composed of small purple particles on both dorsal and ventral body wall. This is the second species of Elpidia Théel, 1876 from Japanese abyssal depths. The diagnosis of the genus Elpidia is modified to distin- guish from all other elpidiid genera. -
Serpentine Volcanoes
2016 Deepwater Exploration of the Marianas Serpentine Volcanoes Focus Serpentine mud volcanoes Grade Level 9-12 (Earth Science) Focus Question What are serpentine mud volcanoes and what geological and chemical processes are involved with their formation? Learning Objectives • Students will describe serpentinization and explain its significance to deep-sea ecosystems. Materials q Copies of Mud Volcano Inquiry Guide, one copy for each student group Audio-Visual Materials q (Optional) Interactive whiteboard Teaching Time One or two 45-minute class periods Seating Arrangement Groups of two to four students Maximum Number of Students 30 Key Words Mariana Arc Serpentine Mud volcano Mariana Trench Serpentinization Peridotite Image captions/credits on Page 2. Tectonic plate 1 www.oceanexplorer.noaa.gov Serpentine Volcanoes - 2016 Grades 9-12 (Earth Science) Background Information NOTE: Explanations and procedures in this lesson are written at a level appropriate to professional educators. In presenting and discussing this material with students, educators may need to adapt the language and instructional approach to styles that are best suited to specific student groups. The Marina Trench is an oceanic trench in the western Pacific Ocean that is formed by the collision of two large pieces of the Earth’s crust known as tectonic plates. These plates are portions of the Earth’s outer crust (the lithosphere) about 5 km thick, as well as the upper 60 - 75 km of the underlying mantle. The plates move on a hot, flexible mantle layer called the asthenosphere, which is several hundred kilometers thick. The Pacific Ocean Basin lies on top of the Pacific Plate. To the east, new crust is formed by magma rising from deep within Images from Page 1 top to bottom: the Earth. -
Deep–Sea Research I
Deep–Sea Research Part I 122 (2017) 81–94 Contents lists available at ScienceDirect Deep–Sea Research I journal homepage: www.elsevier.com/locate/dsri Dynamic benthic megafaunal communities: Assessing temporal variations MARK in structure, composition and diversity at the Arctic deep-sea observatory HAUSGARTEN between 2004 and 2015 ⁎ J. Taylor , T. Krumpen, T. Soltwedel, J. Gutt, M. Bergmann Alfred-Wegener-Institut, Helmholtz-Zentrum für Polar‐ und Meeresforschung, Am Handelshafen 12, D-27570 Bremerhaven, Germany ARTICLE INFO ABSTRACT Keywords: Established in the Fram Strait in 1999, the LTER (Long-Term Ecological Research) observatory HAUSGARTEN Arctic enables us to study ecological changes on the deep Arctic seafloor. Repeated deployments of a towed camera Deep sea system (Ocean Floor Observation System) along the same tracks allowed us to build a time series longer than a Image analysis decade (2004–2015). Here, we present the first time-series results from a northern and the southernmost Epibenthic megafauna station of the observatory (N3 and S3, ~2650 m and 2350 m depth respectively) obtained via the analysis of still Long-term ecological research imagery. We assess temporal variability in community structure, megafaunal densities and diversity, and use a Photo/video system Time series range of biotic factors, environmental sediment parameters and habitat features to explain the patterns observed. There were significant temporal differences in megafaunal abundances, diversity and habitat features at both stations. A particularly high increase in megafaunal abundance was recorded at N3 from 12.08 ( ± 0.39; 2004) individuals m−2 to 35.21 ( ± 0.97; 2007) ind. m−2 alongside a ten-fold increase in (drop-)stones. -
Magma Genesis at Andaman Volcanic Arc Regime, North- Eastern Indian Ocean: Role of Slab-Mantle Interaction
Article 323 by Abhishek Saha*, Abhay V. Mudholkar, and K.A. Kamesh Raju Magma genesis at Andaman volcanic arc regime, North- eastern Indian Ocean: Role of slab-mantle interaction CSIR-National Institute of Oceanography, Dona Paula, Goa- 403004, India; *Corresponding author, E-mail: [email protected]; [email protected] (Received : 29/11/2018; Revised accepted : 23/05/2019) https://doi.org/10.18814/epiiugs/2020/020019 This study reports new petrological and geochemical slab that collectively account for variable extents of ocean-crust-mantle data of submarine volcanic rocks dredged from the interactions, generation of juvenile crust, hydrothermal activity, ore Andaman arc, northeastern Indian Ocean and evaluates mineralization and magmatism (McCulloch and Gamble, 1991; Stern, 2002; Tatsumi, 2005). The elemental fractionation between the their petrogenetic and tectonic implications. The studied subducted oceanic slab and mantle wedge, the different stages of samples exhibit wide range of compositions including subduction from initiation to maturation and associated melt basalts, andesites, dacites and rhyolites depicting BADR generation processes account for the diverse compositional spectra trend of magmatic differentiation. The basalts are of arc magmatism. The diagnostic geochemical features of arc magmas porphyritic and composed of calcic plagioclase including island arc tholeiites (IAT), calc-alkaline basalt-andesite- phenocrysts embedded in the groundmass consisting of dacite-rhyolite (BADR) associations, boninites, arc picrites, siliceous high-Mg basalts (SHMB), adakites, high-Mg andesites (HMA) and plagioclase, clinopyroxene and volcanic glass. Andesite Nb-enriched basalts (NEB) are influenced by the tectonic framework and dacites comprise clusters of plagioclase are along the arc, geometry of the subducting plate, modification and phenocrysts embedded in glassy ground mass depicting enrichment of depleted mantle wedge by influx of materials released glomeroporphyritic and vitrophyric textures. -
How to Cite: Krupnik, D., Flores, J.A., Villarreal
How to cite: Krupnik, D., Flores, J.A., Villarreal, D.P., and Capuano, R.M, 2018, University of Houston, Earth and Atmospheric Sciences, 2018 Student Research Day & Industrial Open House Abstracts, University of Houston Department of Earth and Atmospheric Sciences, 69p. http://www.uh.edu/nsm/earth-atmospheric/news-events/past-events/research-day/2018/final_abstractcompilation_srd2018.pdf © University of Houston, 2018 EDITORS Diana Krupnik completed her BS degree in Biology with a minor in Chemistry in 2012 and subsequently finished a degree in Geology in 2014 at the University of Houston. During her Bachelors, she completed a senior thesis in the field of remote sensing for vegetation studies. Currently, she is working on a PhD in geology, with a research focus in ground-based remote sensing used for detailed outcrop studies. Joshua A. Flores completed a BS in Geology form Brigham Young University in 2013 and then worked with EGI at the University of Utah as a research assistant before beginning his PhD in Geology at the University of Houston. His research focuses on plate triple junctions and their roles in boninite petrogenesis under the direction of John Casey. Dustin P. Villarreal (Student Research Day Committee Chair) received his B.S. in Geology from the University of Houston in 2012 and is currently a PhD candidate in geology under advisor Dr. Alexander Robinson. His research focus is understanding the origin, development, and deformation history of the upper continental crust in both convergent and divergent tectonic settings. His PhD project seeks to understand the Mesozoic history of the Pamir mountains. After graduating, he hopes to apply his geologic interest in understanding basin evolution for oil and gas production. -
GMT Based Comparative Analysis and Geomorphological Mapping of the Kermadec and Tonga Trenches, Southwest Pacific Ocean Polina Lemenkova
GMT Based Comparative Analysis and Geomorphological Mapping of the Kermadec and Tonga Trenches, Southwest Pacific Ocean Polina Lemenkova To cite this version: Polina Lemenkova. GMT Based Comparative Analysis and Geomorphological Mapping of the Ker- madec and Tonga Trenches, Southwest Pacific Ocean. Geographia Technica, Cluj University Press, 2019, 14 (2), pp.39-48. 10.21163/GT_2019.142.04. hal-02333464 HAL Id: hal-02333464 https://hal.archives-ouvertes.fr/hal-02333464 Submitted on 16 Dec 2019 HAL is a multi-disciplinary open access L’archive ouverte pluridisciplinaire HAL, est archive for the deposit and dissemination of sci- destinée au dépôt et à la diffusion de documents entific research documents, whether they are pub- scientifiques de niveau recherche, publiés ou non, lished or not. The documents may come from émanant des établissements d’enseignement et de teaching and research institutions in France or recherche français ou étrangers, des laboratoires abroad, or from public or private research centers. publics ou privés. Distributed under a Creative Commons Attribution| 4.0 International License Geographia Technica, Vol. 14, Issue 2, 2019, pp 39 to 48 GMT BASED COMPARATIVE ANALYSIS AND GEOMORPHOLOGICAL MAPPING OF THE KERMADEC AND TONGA TRENCHES, SOUTHWEST PACIFIC OCEAN Polina LEMENKOVA1 DOI: 10.21163/GT_2019.142.04 ABSTRACT: Current study is focused on the GMT based modelling of the two hadal trenches located in southwest Pacific Ocean, eastwards from Australia: Tonga and Kermadec. Due to its inaccessible location, the seafloor of the deep-sea trench can only be visualized using remote sensing tools and advanced algorithms of data analysis. The importance of the developing and technical improving of the innovative methods in cartographic data processing is indisputable. -
Final Report Form
Appendix K – OSRI Grant Policy Manual Final Report Form - Oil Spill Recovery Institute An electronic copy of this report shall be submitted by mail, or e-mail to the OSRI Research Program Manager [email protected] and Financial Office [email protected] Mailing address: P.O. Box 705 - Cordova, AK 99574 - Deadline for this report: Submittal within 90 days of grant/award expiration. Also, note that a summary Financial Statement shall be submitted within 45 days of the grant expiration. The final invoice and financial statement is due within 90 days of the grant/award expiration. Today’s date: 15 April 2014 Name of awardee/grantee: Bodil Bluhm OSRI Contract Number: 11-10-14 Project title: Data rescue: Epibenthic invertebrates from the Beaufort Sea sampled during WEBSEC and OCS cruises in the 1970s Dates project began and ended: PART I - Outline for Final Program or Technical Report This report must be submitted by all grantees. However, for those whose project work resulted in a peer reviewed publication (whether in draft or final form), this report may be abbreviated and the publication attached as part of the report. A. Non-technical Abstract or summary of project work that does not exceed 2 pages and includes an overview of the project. This abstract should describe the nature and significance of the project. It may be provided to the Advisory Board and could be used by OSRI staff to answer inquiries as to the nature and significance of the project. This project sought to rescue data on epibenthic invertebrates and fish sampled by trawls and photographs in the Alaskan Beaufort Sea during Western Beaufort Sea Ecological Cruise (WEBSEC) and Outer Continental Shelf (OCS) surveys in the 1970s.