Linking Microbial Functioning and Trophic Pathways to Ecological Status in a Coastal Mediterranean Ecosystem
Total Page:16
File Type:pdf, Size:1020Kb
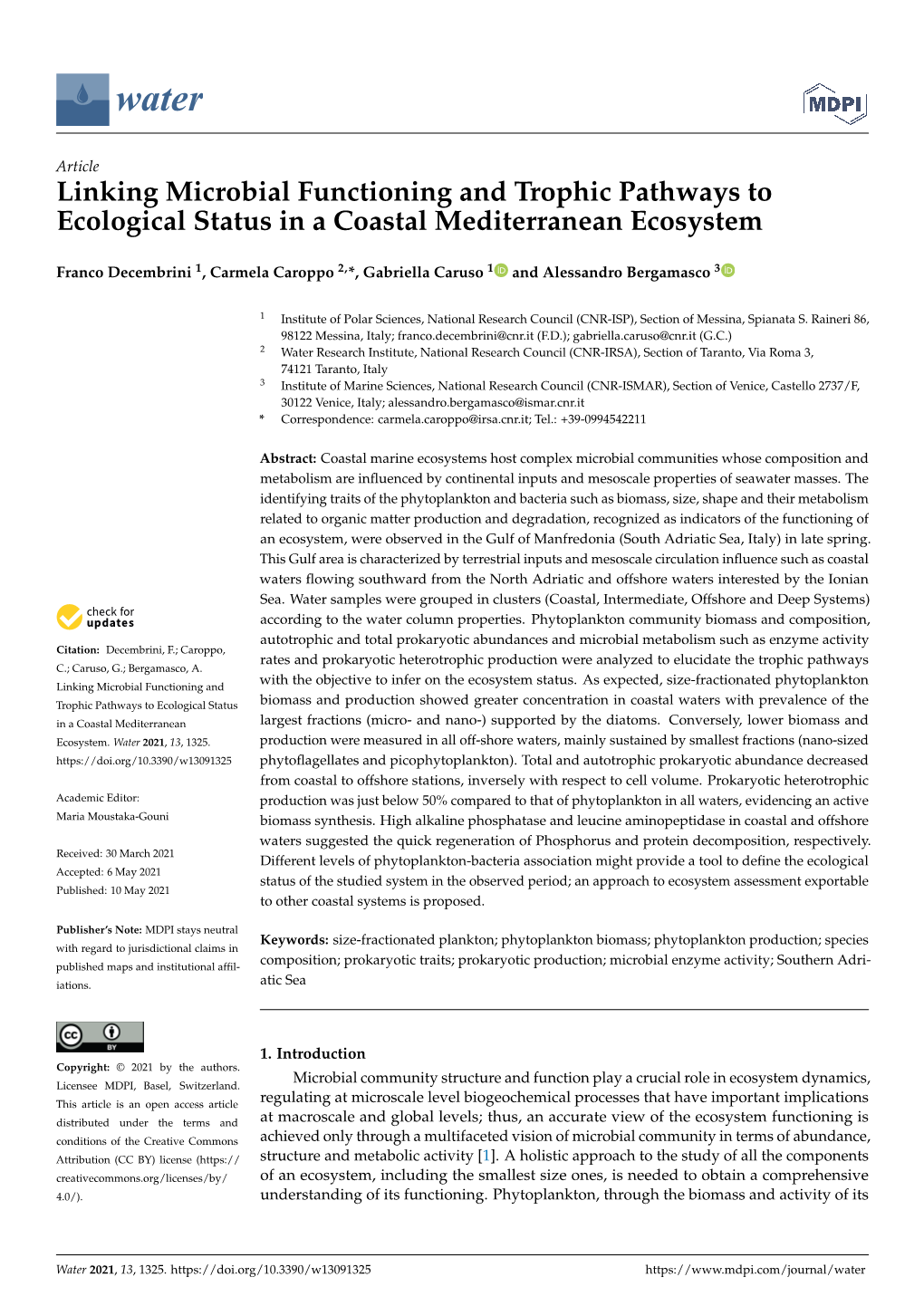
Load more
Recommended publications
-
Biocultural Indicators to Support Locally Led Environmental Management and Monitoring
Copyright © 2019 by the author(s). Published here under license by the Resilience Alliance. DeRoy, B. C., C. T. Darimont, and C. N. Service. 2019. Biocultural indicators to support locally led environmental management and monitoring. Ecology and Society 24(4):21. https://doi.org/10.5751/ES-11120-240421 Synthesis Biocultural indicators to support locally led environmental management and monitoring Bryant C. DeRoy 1,2, Chris T. Darimont 1,2 and Christina N. Service 1,2,3 ABSTRACT. Environmental management (EM) requires indicators to inform objectives and monitor the impacts or efficacy of management practices. One common approach uses “functional ecological” indicators, which are typically species whose presence or abundance are tied to functional ecological processes, such as nutrient productivity and availability, trophic interactions, and habitat connectivity. In contrast, and used for millennia by Indigenous peoples, biocultural indicators are rooted in local values and place- based relationships between nature and people. In many landscapes today where Indigenous peoples are reasserting sovereignty and governance authority over natural resources, the functional ecological approach to indicator development does not capture fundamental values and ties to the natural world that have supported social-ecological systems over the long term. Accordingly, we argue that the development and use of biocultural indicators to shape, monitor, and evaluate the success of EM projects will be critical to achieving ecological and social sustainability today. We have provided a framework composed of criteria to be considered when selecting and applying meaningful and efficacious biocultural indicators among the diverse array of potential species and values. We used a case study from a region now referred to as coastal British Columbia, Canada, to show how the suggested application of functional ecological indicators by the provincial government created barriers to the development of meaningful cogovernance. -
Response of Marine Food Webs to Climate-Induced Changes in Temperature and Inflow of Allochthonous Organic Matter
Response of marine food webs to climate-induced changes in temperature and inflow of allochthonous organic matter Rickard Degerman Department of Ecology and Environmental Science 901 87 Umeå Umeå 2015 1 Copyright©Rickard Degerman ISBN: 978-91-7601-266-6 Front cover illustration by Mats Minnhagen Printed by: KBC Service Center, Umeå University Umeå, Sweden 2015 2 Tillägnad Maria, Emma och Isak 3 Table of Contents Abstract 5 List of papers 6 Introduction 7 Aquatic food webs – different pathways Food web efficiency – a measure of ecosystem function Top predators cause cascade effects on lower trophic levels The Baltic Sea – a semi-enclosed sea exposed to multiple stressors Varying food web structures Climate-induced changes in the marine ecosystem Food web responses to increased temperature Responses to inputs of allochthonous organic matter Objectives 14 Material and Methods 14 Paper I Paper II and III Paper IV Results and Discussion 18 Effect of temperature and nutrient availability on heterotrophic bacteria Influence of food web length and labile DOC on pelagic productivity and FWE Consequences of changes in inputs of ADOM and temperature for pelagic productivity and FWE Control of pelagic productivity, FWE and ecosystem trophic balance by colored DOC Conclusion and future perspectives 21 Author contributions 23 Acknowledgements 23 Thanks 24 References 25 4 Abstract Global records of temperature show a warming trend both in the atmosphere and in the oceans. Current climate change scenarios indicate that global temperature will continue to increase in the future. The effects will however be very different in different geographic regions. In northern Europe precipitation is projected to increase along with temperature. -
A New Type of Plankton Food Web Functioning in Coastal Waters Revealed by Coupling Monte Carlo Markov Chain Linear Inverse Metho
A new type of plankton food web functioning in coastal waters revealed by coupling Monte Carlo Markov Chain Linear Inverse method and Ecological Network Analysis Marouan Meddeb, Nathalie Niquil, Boutheina Grami, Kaouther Mejri, Matilda Haraldsson, Aurélie Chaalali, Olivier Pringault, Asma Sakka Hlaili To cite this version: Marouan Meddeb, Nathalie Niquil, Boutheina Grami, Kaouther Mejri, Matilda Haraldsson, et al.. A new type of plankton food web functioning in coastal waters revealed by coupling Monte Carlo Markov Chain Linear Inverse method and Ecological Network Analysis. Ecological Indicators, Elsevier, 2019, 104, pp.67-85. 10.1016/j.ecolind.2019.04.077. hal-02146355 HAL Id: hal-02146355 https://hal.archives-ouvertes.fr/hal-02146355 Submitted on 3 Jun 2019 HAL is a multi-disciplinary open access L’archive ouverte pluridisciplinaire HAL, est archive for the deposit and dissemination of sci- destinée au dépôt et à la diffusion de documents entific research documents, whether they are pub- scientifiques de niveau recherche, publiés ou non, lished or not. The documents may come from émanant des établissements d’enseignement et de teaching and research institutions in France or recherche français ou étrangers, des laboratoires abroad, or from public or private research centers. publics ou privés. 1 A new type of plankton food web functioning in coastal waters revealed by coupling 2 Monte Carlo Markov Chain Linear Inverse method and Ecological Network Analysis 3 4 5 Marouan Meddeba,b*, Nathalie Niquilc, Boutheïna Gramia,d, Kaouther Mejria,b, Matilda 6 Haraldssonc, Aurélie Chaalalic,e,f, Olivier Pringaultg, Asma Sakka Hlailia,b 7 8 aUniversité de Carthage, Faculté des Sciences de Bizerte, Laboratoire de phytoplanctonologie 9 7021 Zarzouna, Bizerte, Tunisie. -
Modeling Vitamin B1 Transfer to Consumers in the Aquatic Food Web M
www.nature.com/scientificreports OPEN Modeling vitamin B1 transfer to consumers in the aquatic food web M. J. Ejsmond 1,2, N. Blackburn3, E. Fridolfsson 2, P. Haecky3, A. Andersson4,5, M. Casini 6, A. Belgrano6,7 & S. Hylander2 Received: 10 January 2019 Vitamin B1 is an essential exogenous micronutrient for animals. Mass death and reproductive failure in Accepted: 26 June 2019 top aquatic consumers caused by vitamin B1 defciency is an emerging conservation issue in Northern Published: xx xx xxxx hemisphere aquatic ecosystems. We present for the frst time a model that identifes conditions responsible for the constrained fow of vitamin B1 from unicellular organisms to planktivorous fshes. The fow of vitamin B1 through the food web is constrained under anthropogenic pressures of increased nutrient input and, driven by climatic change, increased light attenuation by dissolved substances transported to marine coastal systems. Fishing pressure on piscivorous fsh, through increased abundance of planktivorous fsh that overexploit mesozooplankton, may further constrain vitamin B1 fow from producers to consumers. We also found that key ecological contributors to the constrained fow of vitamin B1 are a low mesozooplankton biomass, picoalgae prevailing among primary producers and low fuctuations of population numbers of planktonic organisms. Vitamin B1 (thiamin) is necessary for the proper functioning of the majority of organisms because it serves as a cofactor that associates with a number of enzymes involved in primary carbohydrate and amino acid metabo- 1 2 lism . Vitamin B1 defciency compromises mitochondrial functioning and causes nerve signaling malfunction 3 in animals . On a systemic level, low vitamin B1 levels translate into impaired health, immunosuppression and 4 reproductive failures . -
Selph Et Al-2021.Pdf
Journal of Plankton Research academic.oup.com/plankt Downloaded from https://academic.oup.com/plankt/advance-article/doi/10.1093/plankt/fbab006/6161505 by Florida State University Law Library user on 10 March 2021 J. Plankton Res. (2021) 1–20. doi:10.1093/plankt/fbab006 BLOOFINZ - Gulf of Mexico Phytoplankton community composition and biomass in the oligotrophic Gulf of Mexico KAREN E. SELPH1,*, RASMUS SWALETHORP2, MICHAEL R. STUKEL3,THOMASB.KELLY3, ANGELA N. KNAPP3, KELSEY FLEMING2, TABITHA HERNANDEZ2 AND MICHAEL R. LANDRY2 1department of oceanography, university of hawaii at manoa, 1000 pope road, honolulu, hi 96822, usa, 2scripps institution of oceanography, 9500 gilman drive, la jolla, ca 92093-0227, usa and 3department of earth, ocean and atmospheric science, florida state university, tallahassee, fl 32306, usa *corresponding author: [email protected] Received October 13, 2020; editorial decision January 10, 2021; accepted January 10, 2021 Corresponding editor: Lisa Campbell Biomass and composition of the phytoplankton community were investigated in the deep-water Gulf of Mexico (GoM) at the edges of Loop Current anticyclonic eddies during May 2017 and May 2018. Using flow cytometry, high-performance liquid chromatography pigments and microscopy, we found euphotic zone integrated chlorophyll aof ∼10 mg m−2 and autotrophic carbon ranging from 463 to 1268 mg m−2, dominated by picoplankton (<2μm cells). Phytoplankton assemblages were similar to the mean composition at the Bermuda Atlantic Time-series Study site, but differed from the Hawaii Ocean Times-series site. GoM phytoplankton biomass was ∼2-fold higher at the deep chlorophyll maximum (DCM) relative to the mixed layer (ML). Prochlorococcus and prymnesiophytes were the dominant taxa throughout the euphotic zone; however, other eukaryotic taxa had significant biomass in the DCM. -
Microbial Loop' in Stratified Systems
MARINE ECOLOGY PROGRESS SERIES Vol. 59: 1-17, 1990 Published January 11 Mar. Ecol. Prog. Ser. 1 A steady-state analysis of the 'microbial loop' in stratified systems Arnold H. Taylor, Ian Joint Plymouth Marine Laboratory, Prospect Place, West Hoe, Plymouth PLl 3DH, United Kingdom ABSTRACT. Steady state solutions are presented for a simple model of the surface mixed layer, which contains the components of the 'microbial loop', namely phytoplankton, picophytoplankton, bacterio- plankton, microzooplankton, dissolved organic carbon, detritus, nitrate and ammonia. This system is assumed to be in equilibrium with the larger grazers present at any time, which are represented as an external mortality function. The model also allows for dissolved organic nitrogen consumption by bacteria, and self-grazing and mixotrophy of the microzooplankton. The model steady states are always stable. The solution shows a number of general properties; for example, biomass of each individual component depends only on total nitrogen concentration below the mixed layer, not whether the nitrogen is in the form of nitrate or ammonia. Standing stocks and production rates from the model are compared with summer observations from the Celtic Sea and Porcupine Sea Bight. The agreement is good and suggests that the system is often not far from equilibrium. A sensitivity analysis of the model is included. The effect of varying the mixing across the pycnocline is investigated; more intense mixing results in the large phytoplankton population increasing at the expense of picophytoplankton, micro- zooplankton and DOC. The change from phytoplankton to picophytoplankton dominance at low mixing occurs even though the same physiological parameters are used for both size fractions. -
Developments in Aquatic Microbiology
INTERNATL MICROBIOL (2000) 3: 203–211 203 © Springer-Verlag Ibérica 2000 REVIEW ARTICLE Samuel P. Meyers Developments in aquatic Department of Oceanography and Coastal Sciences, Louisiana State University, microbiology Baton Rouge, LA, USA Received 30 August 2000 Accepted 29 September 2000 Summary Major discoveries in marine microbiology over the past 4-5 decades have resulted in the recognition of bacteria as a major biomass component of marine food webs. Such discoveries include chemosynthetic activities in deep-ocean ecosystems, survival processes in oligotrophic waters, and the role of microorganisms in food webs coupled with symbiotic relationships and energy flow. Many discoveries can be attributed to innovative methodologies, including radioisotopes, immunofluores- cent-epifluorescent analysis, and flow cytometry. The latter has shown the key role of marine viruses in marine system energetics. Studies of the components of the “microbial loop” have shown the significance of various phagotrophic processes involved in grazing by microinvertebrates. Microbial activities and dissolved organic carbon are closely coupled with the dynamics of fluctuating water masses. New biotechnological approaches and the use of molecular biology techniques still provide new and relevant information on the role of microorganisms in oceanic and estuarine environments. International interdisciplinary studies have explored ecological aspects of marine microorganisms and their significance in biocomplexity. Studies on the Correspondence to: origins of both life and ecosystems now focus on microbiological processes in the Louisiana State University Station. marine environment. This paper describes earlier and recent discoveries in marine Post Office Box 19090-A. Baton Rouge, LA 70893. USA (aquatic) microbiology and the trends for future work, emphasizing improvements Tel.: +1-225-3885180 in methodology as major catalysts for the progress of this broadly-based field. -
Ascendency As Ecological Indicator for Environmental Quality Assessment at the Ecosystem Level: a Case Study
Hydrobiologia (2006) 555:19–30 Ó Springer 2006 H. Queiroga, M.R. Cunha, A. Cunha, M.H. Moreira, V. Quintino, A.M. Rodrigues, J. Seroˆ dio & R.M. Warwick (eds), Marine Biodiversity: Patterns and Processes, Assessment, Threats, Management and Conservation DOI 10.1007/s10750-005-1102-8 Ascendency as ecological indicator for environmental quality assessment at the ecosystem level: a case study J. Patrı´ cio1,*, R. Ulanowicz2, M. A. Pardal1 & J. C. Marques1 1IMAR- Institute of Marine Research, Department of Zoology, Faculty of Sciences and Technology, University of Coimbra, 3004-517, Coimbra, Portugal 2Chesapeake Biological Laboratory, Center for Environmental and Estuarine Studies, University of Maryland, Solomons, Maryland, 20688-0038, USA (*Author for correspondence: E-mail: [email protected]) Key words: network analysis, ascendency, eutrophication, estuary Abstract Previous studies have shown that when an ecosystem consists of many interacting components it becomes impossible to understand how it functions by focussing only on individual relationships. Alternatively, one can attempt to quantify system behaviour as a whole by developing ecological indicators that combine numerous environmental factors into a single value. One such holistic measure, called the system ‘ascen- dency’, arises from the analysis of networks of trophic exchanges. It deals with the joint quantification of overall system activity with the organisation of the component processes and can be used specifically to identify the occurrence of eutrophication. System ascendency analyses were applied to data over a gradient of eutrophication in a well documented small temperate intertidal estuary. Three areas were compared along the gradient, respectively, non eutrophic, intermediate eutrophic, and strongly eutrophic. Values of other measures related to the ascendency, such as the total system throughput, development capacity, and average mutual information, as well as the ascendency itself, were clearly higher in the non-eutrophic area. -
Diversity and Evolution of Bacterial Bioluminescence Genes in the Global Ocean Thomas Vannier, Pascal Hingamp, Floriane Turrel, Lisa Tanet, Magali Lescot, Y
Diversity and evolution of bacterial bioluminescence genes in the global ocean Thomas Vannier, Pascal Hingamp, Floriane Turrel, Lisa Tanet, Magali Lescot, Y. Timsit To cite this version: Thomas Vannier, Pascal Hingamp, Floriane Turrel, Lisa Tanet, Magali Lescot, et al.. Diversity and evolution of bacterial bioluminescence genes in the global ocean. NAR Genomics and Bioinformatics, Oxford University Press, 2020, 2 (2), 10.1093/nargab/lqaa018. hal-02514159 HAL Id: hal-02514159 https://hal.archives-ouvertes.fr/hal-02514159 Submitted on 21 Mar 2020 HAL is a multi-disciplinary open access L’archive ouverte pluridisciplinaire HAL, est archive for the deposit and dissemination of sci- destinée au dépôt et à la diffusion de documents entific research documents, whether they are pub- scientifiques de niveau recherche, publiés ou non, lished or not. The documents may come from émanant des établissements d’enseignement et de teaching and research institutions in France or recherche français ou étrangers, des laboratoires abroad, or from public or private research centers. publics ou privés. Published online 14 March 2020 NAR Genomics and Bioinformatics, 2020, Vol. 2, No. 2 1 doi: 10.1093/nargab/lqaa018 Diversity and evolution of bacterial bioluminescence genes in the global ocean Thomas Vannier 1,2,*, Pascal Hingamp1,2, Floriane Turrel1, Lisa Tanet1, Magali Lescot 1,2,* and Youri Timsit 1,2,* 1Aix Marseille Univ, Universite´ de Toulon, CNRS, IRD, MIO UM110, 13288 Marseille, France and 2Research / Federation for the study of Global Ocean Systems Ecology and Evolution, FR2022 Tara GOSEE, 3 rue Michel-Ange, Downloaded from https://academic.oup.com/nargab/article-abstract/2/2/lqaa018/5805306 by guest on 21 March 2020 75016 Paris, France Received October 21, 2019; Revised February 14, 2020; Editorial Decision March 02, 2020; Accepted March 06, 2020 ABSTRACT ganisms and is particularly widespread in marine species (7–9). -
ECOLOGICAL FOOTPRINT AN]) Appropriathd CARRYING CAPACITY: a TOOL for PLANNING TOWARD SUSTAINABILITY
ECOLOGICAL FOOTPRINT AN]) APPROPRIAThD CARRYING CAPACITY: A TOOL FOR PLANNING TOWARD SUSTAINABILITY by MATHIS WACKERNAGEL Dip!. Ing., The Swiss Federal Institute of Technology, ZUrich, 1988 A THESIS SUBMITTED IN PARTIAL FULFILLMENT OF THE REQUIREMENTS FOR THE DEGREE OF DOCTOR OF PHILOSOPHY in THE FACULTY OF GRADUATE STUDIES (School of Community and Regional Planning) We accept this thesis as conforming to the r ired standard THE UNIVERSITY OF BRITISH COLUMBIA October 1994 © Mathis Wackernagel, 1994 advanced In presenting this thesis in partial fulfilment of the requirements for an Library shall make it degree at the University of British Columbia, I agree that the that permission for extensive freely available for reference and study. I further agree copying of this thesis for scholarly purposes may be granted by the head of my department or by his or her representatives. It is understood that copying or publication of this thesis for financial gain shall not be allowed without my written permission. (Signature) ejb’i’t/ Pios-ii’ii &toof of C iwivry Gf (i l r€dva k hi di’e The University of British Columbia Vancouver, Canada Date O 6) ) DE-6 (2/88) ABSThACT There is mounting evidence that the ecosystems of Earth cannot sustain current levels of economic activity, let alone increased levels. Since some consume Earth’s resources at a rate that will leave little for future generations, while others still live in debilitating poverty, the UN’s World Commission on Environment and Economic Development has called for development that is sustainable. The purpose of this thesis is to further develop and test a planning tool that can assist in translating the concern about the sustainability crisis into public action. -
The Effects of Eutrophication on the Structure and Function of Microbial Biofilms
The Effects of Eutrophication on the Structure and Function of Microbial Biofilms David J. Van Horn1*, Cliff N. Dahm1 1UNM Department of Biology, 167 Castetter Hall MSC03 2020, 1 University of New Mexico, Albuquerque, NM 87131-0001 Abstract: Biofilms are the dominate form of microbial life in aquatic ecosystems and are responsible for performing a wide variety of ecosystem services including nutrient and organic matter processing and retention. Understanding how eutrophication impacts these communities is essential for ecosystem managers as many aquatic ecosystems are being enriched by anthropogenic activities. This study investigated the effects of eutrophication on biofilm productivity, community structure and diversity, and function. Increasing background dissolved organic carbon and nutrient concentrations by a factor of twelve increased biofilm ash free dry mass and the abundance of live cells ~ 22 and 200 fold respectively. Extracellular enzyme activities for five enzymes from the same samples showed a range of increase from ~ 300 times for phosphatase to 8000 times for N-acetylglucosaminidase. Enrichment decreased the bacterial diversity of these biofilms and resulted in a gradual shift in community structure that intensified from low to high enrichment. These data indicate that the productivity, community structure, and function of stream biofilm communities are highly responsive to eutrophication inputs. 1 Introduction: Microbes are the most abundant organisms on Earth and play a central role in all global processes. While often overlooked due to their inconspicuous nature, microbes contain as much carbon and ten times more nitrogen and phosphorus than plants (Whitman et al. 1998), possess unique metabolic pathways essential to biogeochemical cycles (Schlesinger 1997), and represent a potentially massive pool of genetic diversity that is only now being explored (Curtis et al. -
Controls and Structure of the Microbial Loop
Controls and Structure of the Microbial Loop A symposium organized by the Microbial Oceanography summer course sponsored by the Agouron Foundation Saturday, July 1, 2006 Asia Room, East-West Center, University of Hawaii Symposium Speakers: Peter J. leB Williams (University of Bangor, Wales) David L. Kirchman (University of Delaware) Daniel J. Repeta (Woods Hole Oceanographic Institute) Grieg Steward (University of Hawaii) The oceans constitute the largest ecosystems on the planet, comprising more than 70% of the surface area and nearly 99% of the livable space on Earth. Life in the oceans is dominated by microbes; these small, singled-celled organisms constitute the base of the marine food web and catalyze the transformation of energy and matter in the sea. The microbial loop describes the dynamics of microbial food webs, with bacteria consuming non-living organic matter and converting this energy and matter into living biomass. Consumption of bacteria by predation recycles organic matter back into the marine food web. The speakers of this symposium will explore the processes that control the structure and functioning of microbial food webs and address some of these fundamental questions: What aspects of microbial activity do we need to measure to constrain energy and material flow into and out of the microbial loop? Are we able to measure bacterioplankton dynamics (biomass, growth, production, respiration) well enough to edu/agouroninstitutecourse understand the contribution of the microbial loop to marine systems? What factors control the flow of material and energy into and out of the microbial loop? At what scales (space and time) do we need to measure processes controlling the growth and metabolism of microorganisms? How does our knowledge of microbial community structure and diversity influence our understanding of the function of the microbial loop? Program: 9:00 am Welcome and Introductory Remarks followed by: Peter J.