A New Biophysical Decompression Model for Estimating the Risk of Articular Bends During and After Decompression J
Total Page:16
File Type:pdf, Size:1020Kb
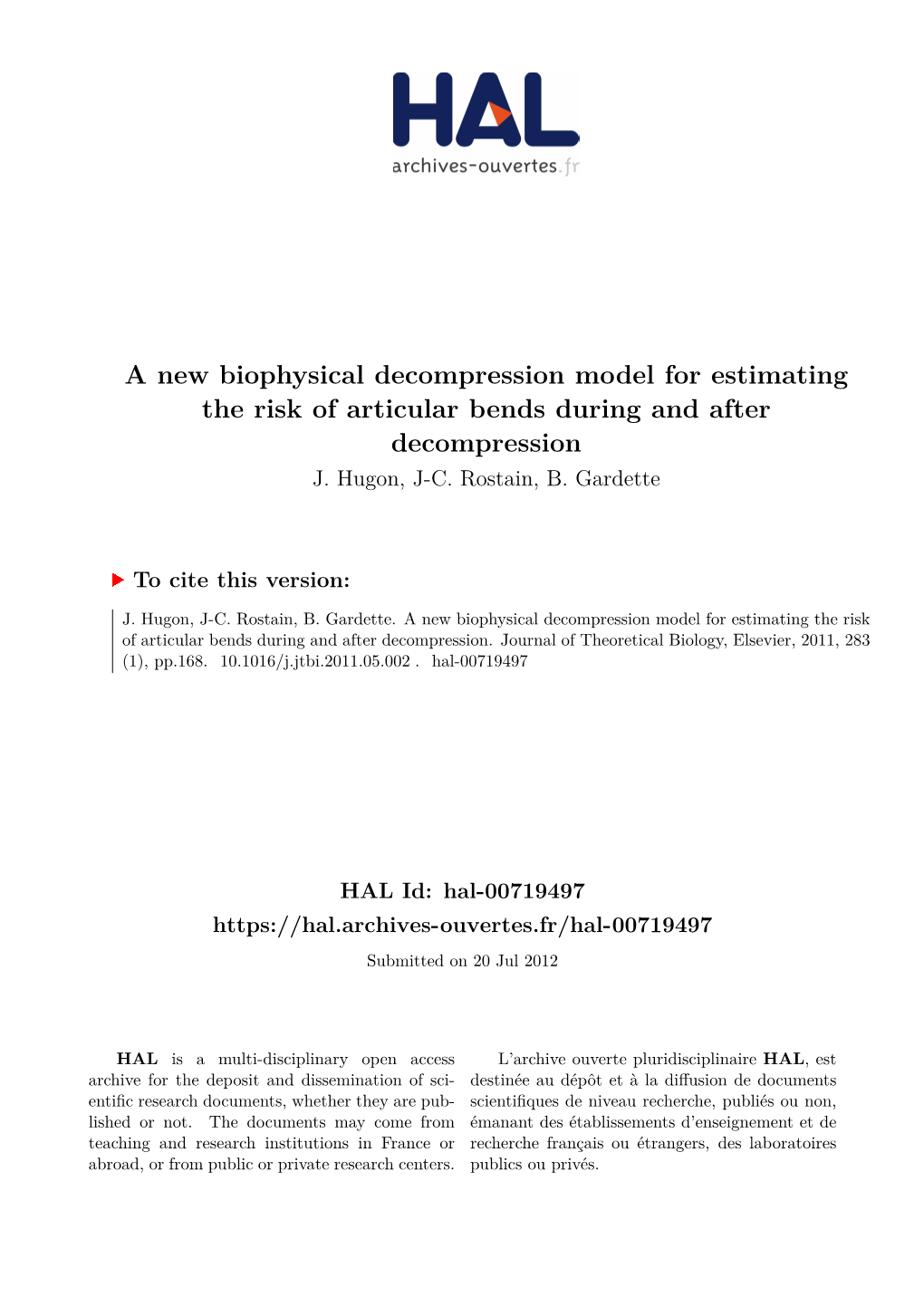
Load more
Recommended publications
-
Full Paper/Talk Deep Stops and Shallow Stops – Fact and Fancy
Full Paper/Talk Deep Stops and Shallow Stops – Fact and Fancy B.R. Wienke1 and T.R. O’Leary2 1 C&C Dive Team Ldr, Program Manager Computational Physics, LANL, MS D490, Los Alamos, NM 87545 [email protected] 2 Director, NAUI Technical Diving Operations, 33256 State Rd 100, South Padre Island, TX, 78597 Abstract The question of deep stops and shallow stops is interesting and fraught with controversy in diving circles and operations, training, exploration and scientific endeavors. Plus frought with some misunderstanding which is understandable as the issues are complex. We therefore attempt a short history of deep and shallow stops, physical aspects, staging differences, diving tests, models with data correlations and data banks with user statistics and DCS outcomes as diver amplification. Pros and cons of both deep stop and shallow stop staging are presented. Misfacts are righted when appropriate. Chamber, wet and Doppler tests are contrasted. A compendium of Training Agency Standards regarding deep and shallow stops is included. Dive software is also detailed. Some commercial diving operations are discussed. A short tabulation of dive computer and software algorithms is given. From diving data, tests, DCS outcomes and field usage, we conclude that both deep stops and shallow stops are safely employed in recreational and technical diving. That is a good thing but choose your deco wisely and know why. Keywords: computational models, decompression staging, profile data, risk, statistical correlations, tests Acronyms and Nomenclature ANDI: Association of Nitrox Diving Instructors. BM: bubble phase model dividing the body into tissue compartments with halftimes that are coupled to inert gas diffusion across bubble film surfaces of exponential size distribution constrained in cumulative growth by a volume limit point. -
Deadly Acute Decompression Sickness in Risso's Dolphins
www.nature.com/scientificreports OPEN Deadly acute Decompression Sickness in Risso’s dolphins A. Fernández, E. Sierra, J. Díaz-Delgado, S. Sacchini , Y. Sánchez-Paz, C. Suárez-Santana, M. Arregui, M. Arbelo & Y. Bernaldo de Quirós Received: 19 April 2017 Diving air-breathing vertebrates have long been considered protected against decompression sickness Accepted: 5 October 2017 (DCS) through anatomical, physiological, and behavioural adaptations. However, an acute systemic gas Published: xx xx xxxx and fat embolic syndrome similar to DCS in human divers was described in beaked whales that stranded in temporal and spatial association with military exercises involving high-powered sonar. More recently, DCS has been diagnosed in bycaught sea turtles. Both cases were linked to human activities. Two Risso’s dolphin (Grampus griseus) out of 493 necropsied cetaceans stranded in the Canary Islands in a 16-year period (2000–2015), had a severe acute decompression sickness supported by pathological fndings and gas analysis. Deadly systemic, infammatory, infectious, or neoplastic diseases, ship collision, military sonar, fsheries interaction or other type of lethal inducing associated trauma were ruled out. Struggling with a squid during hunting is discussed as the most likely cause of DCS. Pathologies related to efects of changes in pressure are well known among human divers. Decompression sick- ness (DCS) is a syndrome related to the formation of gas bubbles in blood and/or tissues when the sum of the dissolved gas tensions exceeds the local absolute pressure. Gas bubbles may have biochemical efects and disrupt the tissues or occlude the vessels with clinical and pathological signs and, in certain cases, death1. -
Dive Theory Guide
DIVE THEORY STUDY GUIDE by Rod Abbotson CD69259 © 2010 Dive Aqaba Guidelines for studying: Study each area in order as the theory from one subject is used to build upon the theory in the next subject. When you have completed a subject, take tests and exams in that subject to make sure you understand everything before moving on. If you try to jump around or don’t completely understand something; this can lead to gaps in your knowledge. You need to apply the knowledge in earlier sections to understand the concepts in later sections... If you study this way you will retain all of the information and you will have no problems with any PADI dive theory exams you may take in the future. Before completing the section on decompression theory and the RDP make sure you are thoroughly familiar with the RDP, both Wheel and table versions. Use the appropriate instructions for use guides which come with the product. Contents Section One PHYSICS ………………………………………………page 2 Section Two PHYSIOLOGY………………………………………….page 11 Section Three DECOMPRESSION THEORY & THE RDP….……..page 21 Section Four EQUIPMENT……………………………………………page 27 Section Five SKILLS & ENVIRONMENT…………………………...page 36 PHYSICS SECTION ONE Light: The speed of light changes as it passes through different things such as air, glass and water. This affects the way we see things underwater with a diving mask. As the light passes through the glass of the mask and the air space, the difference in speed causes the light rays to bend; this is called refraction. To the diver wearing a normal diving mask objects appear to be larger and closer than they actually are. -
Biomechanics of Safe Ascents Workshop
PROCEEDINGS OF BIOMECHANICS OF SAFE ASCENTS WORKSHOP — 10 ft E 30 ft TIME AMERICAN ACADEMY OF UNDERWATER SCIENCES September 25 - 27, 1989 Woods Hole, Massachusetts Proceedings of the AAUS Biomechanics of Safe Ascents Workshop Michael A. Lang and Glen H. Egstrom, (Editors) Copyright © 1990 by AMERICAN ACADEMY OF UNDERWATER SCIENCES 947 Newhall Street Costa Mesa, CA 92627 All Rights Reserved No part of this book may be reproduced in any form by photostat, microfilm, or any other means, without written permission from the publishers Copies of these Proceedings can be purchased from AAUS at the above address This workshop was sponsored in part by the National Oceanic and Atmospheric Administration (NOAA), Department of Commerce, under grant number 40AANR902932, through the Office of Undersea Research, and in part by the Diving Equipment Manufacturers Association (DEMA), and in part by the American Academy of Underwater Sciences (AAUS). The U.S. Government is authorized to produce and distribute reprints for governmental purposes notwithstanding the copyright notation that appears above. Opinions presented at the Workshop and in the Proceedings are those of the contributors, and do not necessarily reflect those of the American Academy of Underwater Sciences PROCEEDINGS OF THE AMERICAN ACADEMY OF UNDERWATER SCIENCES BIOMECHANICS OF SAFE ASCENTS WORKSHOP WHOI/MBL Woods Hole, Massachusetts September 25 - 27, 1989 MICHAEL A. LANG GLEN H. EGSTROM Editors American Academy of Underwater Sciences 947 Newhall Street, Costa Mesa, California 92627 U.S.A. An American Academy of Underwater Sciences Diving Safety Publication AAUSDSP-BSA-01-90 CONTENTS Preface i About AAUS ii Executive Summary iii Acknowledgments v Session 1: Introductory Session Welcoming address - Michael A. -
Part Two Physical Processes in Oceanography
Part Two Physical Processes in Oceanography 8 8.1 Introduction Small-Scale Forty years ago, the detailed physical mechanisms re- Mixing Processes sponsible for the mixing of heat, salt, and other prop- erties in the ocean had hardly been considered. Using profiles obtained from water-bottle measurements, and J. S. Turner their variations in time and space, it was deduced that mixing must be taking place at rates much greater than could be accounted for by molecular diffusion. It was taken for granted that the ocean (because of its large scale) must be everywhere turbulent, and this was sup- ported by the observation that the major constituents are reasonably well mixed. It seemed a natural step to define eddy viscosities and eddy conductivities, or mix- ing coefficients, to relate the deduced fluxes of mo- mentum or heat (or salt) to the mean smoothed gra- dients of corresponding properties. Extensive tables of these mixing coefficients, KM for momentum, KH for heat, and Ks for salinity, and their variation with po- sition and other parameters, were published about that time [see, e.g., Sverdrup, Johnson, and Fleming (1942, p. 482)]. Much mathematical modeling of oceanic flows on various scales was (and still is) based on simple assumptions about the eddy viscosity, which is often taken to have a constant value, chosen to give the best agreement with the observations. This approach to the theory is well summarized in Proudman (1953), and more recent extensions of the method are described in the conference proceedings edited by Nihoul 1975). Though the preoccupation with finding numerical values of these parameters was not in retrospect always helpful, certain features of those results contained the seeds of many later developments in this subject. -
Training Objectives for a Diving Medical Physician
The Diving Medical Advisory Committee Training Objectives for a Diving Medicine Physician This guidance includes all the training objectives agreed by the Diving Medical Advisory Committee, the European Diving Technology Committee and the European Committee for Hyperbaric Medicine in 2011. Rev 1 - 2013 INTRODUCTION The purpose of this document is to define more closely the training objectives in diving physiology and medicine that need to be met by doctors already fully accredited or board-certified in a clinical speciality to national standards. It is based on topic headings that were originally prepared for a working group of European Diving Technology Committee (EDTC) and the European Committee of Hyperbaric Medicine (ECHM) as a guide for diving medicine some 20 years ago by J.Desola (Spain), T.Nome (Norway) & D.H.Elliott (U.K.). The training now required for medical examiners of working divers and for specialist diving medicine physicians was based on a EDTC/ECHM standard 1999 and subsequently has been enhanced by the Diving Medical Advisory Committee (DMAC), revised and agreed in principle by DMAC, EDTC and ECHM in 2010 and then ratified by EDTC and ECHM in 2011. The requirements now relate to an assessment of competence, the need for some training in occupational medicine, the need for maintenance of those skills by individual ‘refresher training’. Formal recognition of all this includes the need to involve a national authority for medical education. These objectives have been applied internationally to doctors who provide medical support to working divers. (Most recreational instructors and dive guides are, by their employment, working divers and so the guidance includes the relevant aspects of recreational diving. -
Diffusion at Work
or collective redistirbution of any portion of this article by photocopy machine, reposting, or other means is permitted only with the approval of The Oceanography Society. Send all correspondence to: [email protected] ofor Th e The to: [email protected] Oceanography approval Oceanography correspondence POall Box 1931, portionthe Send Society. Rockville, ofwith any permittedUSA. articleonly photocopy by Society, is MD 20849-1931, of machine, this reposting, means or collective or other redistirbution article has This been published in hands - on O ceanography Oceanography Diffusion at Work , Volume 20, Number 3, a quarterly journal of The Oceanography Society. Copyright 2007 by The Oceanography Society. All rights reserved. Permission is granted to copy this article for use in teaching and research. Republication, systemmatic reproduction, reproduction, Republication, systemmatic research. for this and teaching article copy to use in reserved.by The 2007 is rights ofAll granted journal Copyright Oceanography The Permission 20, NumberOceanography 3, a quarterly Society. Society. , Volume An Interactive Simulation B Y L ee K arp-B oss , E mmanuel B oss , and J ames L oftin PURPOSE OF ACTIVITY or small particles due to their random (Brownian) motion and The goal of this activity is to help students better understand the resultant net migration of material from regions of high the nonintuitive concept of diffusion and introduce them to a concentration to regions of low concentration. Stirring (where variety of diffusion-related processes in the ocean. As part of material gets stretched and folded) expands the area available this activity, students also practice data collection and statisti- for diffusion to occur, resulting in enhanced mixing compared cal analysis (e.g., average, variance, and probability distribution to that due to molecular diffusion alone. -
A Primer for Technical Diving Decompression Theory
SCUBA AA PPRRIIMMEERR FFOORR TECH TTEECCHHNNIICCAALL DDIIVVIINNGG DDEECCOOMMPPRREESSSSIIOONN PHILIPPINES TTHHEEOORRYY 1 | P a g e ©Andy Davis 2015 www.scubatechphilippines.com Sidemount, Technical & Wreck Guide | Andy Davis First Published 2016 All documents compiled in this publication are open-source and freely available on the internet. Copyright Is applicable to the named authors stated within the document. Cover and logo images are copyright to ScubaTechPhilippines/Andy Davis. Not for resale. This publication is not intended to be used as a substitute for appropriate dive training. Diving is a dangerous sport and proper training should only be conducted under the safe supervision of an appropriate, active, diving instructor until you are fully qualified, and then, only in conditions and circumstances which are as good or better than the conditions in which you were trained. Technical scuba diving should be taught by a specialized instructor with training credentials and experience at that level of diving. Careful risk assessment, continuing education and skill practice may reduce your likelihood of an accident, but are in no means a guarantee of complete safety. This publication assumes a basic understanding of diving skills and knowledge. It should be used to complement the undertaking of prerequisite training on the route to enrolling upon technical diving training. 2 | P a g e ©Andy Davis 2015 www.scubatechphilippines.com This primer on decompression theory is designed as a supplement to your technical diving training. Becoming familiar with the concepts and terms outlined in this document will enable you to get the most out of your theory training with me; and subsequently enjoy safer, more refined dive planning and management in your technical diving activities. -
What Is the Difference Between Osmosis and Diffusion?
What is the difference between osmosis and diffusion? Students are often asked to explain the similarities and differences between osmosis and diffusion or to compare and contrast the two forms of transport. To answer the question, you need to know the definitions of osmosis and diffusion and really understand what they mean. Osmosis And Diffusion Definitions Osmosis: Osmosis is the movement of solvent particles across a semipermeable membrane from a dilute solution into a concentrated solution. The solvent moves to dilute the concentrated solution and equalize the concentration on both sides of the membrane. Diffusion: Diffusion is the movement of particles from an area of higher concentration to lower concentration. The overall effect is to equalize concentration throughout the medium. Osmosis And Diffusion Examples Examples of Osmosis: Examples of osmosis include red blood cells swelling up when exposed to fresh water and plant root hairs taking up water. To see an easy demonstration of osmosis, soak gummy candies in water. The gel of the candies acts as a semipermeable membrane. Examples of Diffusion: Examples of diffusion include perfume filling a whole room and the movement of small molecules across a cell membrane. One of the simplest demonstrations of diffusion is adding a drop of food coloring to water. Although other transport processes do occur, diffusion is the key player. Osmosis And Diffusion Similarities Osmosis and diffusion are related processes that display similarities. Both osmosis and diffusion equalize the concentration of two solutions. Both diffusion and osmosis are passive transport processes, which means they do not require any input of extra energy to occur. -
Gas Exchange and Respiratory Function
LWBK330-4183G-c21_p484-516.qxd 23/07/2009 02:09 PM Page 484 Aptara Gas Exchange and 5 Respiratory Function Applying Concepts From NANDA, NIC, • Case Study and NOC A Patient With Impaired Cough Reflex Mrs. Lewis, age 77 years, is admitted to the hospital for left lower lobe pneumonia. Her vital signs are: Temp 100.6°F; HR 90 and regular; B/P: 142/74; Resp. 28. She has a weak cough, diminished breath sounds over the lower left lung field, and coarse rhonchi over the midtracheal area. She can expectorate some sputum, which is thick and grayish green. She has a history of stroke. Secondary to the stroke she has impaired gag and cough reflexes and mild weakness of her left side. She is allowed food and fluids because she can swallow safely if she uses the chin-tuck maneuver. Visit thePoint to view a concept map that illustrates the relationships that exist between the nursing diagnoses, interventions, and outcomes for the patient’s clinical problems. LWBK330-4183G-c21_p484-516.qxd 23/07/2009 02:09 PM Page 485 Aptara Nursing Classifications and Languages NANDA NIC NOC NURSING DIAGNOSES NURSING INTERVENTIONS NURSING OUTCOMES INEFFECTIVE AIRWAY CLEARANCE— RESPIRATORY MONITORING— Return to functional baseline sta- Inability to clear secretions or ob- Collection and analysis of patient tus, stabilization of, or structions from the respiratory data to ensure airway patency improvement in: tract to maintain a clear airway and adequate gas exchange RESPIRATORY STATUS: AIRWAY PATENCY—Extent to which the tracheobronchial passages remain open IMPAIRED GAS -
Package 'Scuba'
Package ‘scuba’ May 14, 2021 Version 1.11-0 Date 2021-05-14 Title Diving Calculations and Decompression Models Author Adrian Baddeley [aut, cre], Vittorio Broglio [ctb, dtc], Pedro Antonio Neves [ctb, dtc], Andrew Bassom [ctb], Peter Buzzacott [ctb] Maintainer Adrian Baddeley <[email protected]> Depends R (>= 3.5.0) Imports utils, graphics, stats Description Code for describing and manipulating scuba diving profiles (depth-time curves) and decompression models, for calculating the predictions of decompression models, for calculating maximum no-decompression time and decompression tables, and for performing mixed gas calculations. License GPL (>= 2) LazyData true ByteCompile true NeedsCompilation yes Repository CRAN Date/Publication 2021-05-14 11:20:02 UTC R topics documented: scuba-package . .2 air..............................................7 ascent . .8 baron . .9 bestdoubledive . 10 Bookspan . 12 1 2 scuba-package BuehlmannL16A . 13 chop.dive . 14 deco.ceiling . 15 deepmine . 17 depths.dive . 18 descent . 19 dive ............................................. 20 durations.dive . 23 ead.............................................. 24 END............................................. 25 haldane . 26 hm.............................................. 30 is.nitrox . 32 maxmix . 33 Mmix ............................................ 34 mod............................................. 36 ndl.............................................. 37 nitrox . 38 oxtox . 39 param . 41 pedro . 42 pickmodel . 43 plot.dive . 44 ppO2 ............................................ 46 print.dive . 47 saturated.state . 48 scuba.constants . 49 scuba.disclaimer . 49 showstates . 50 tanklist . 51 times.dive . 52 trimix . 53 whichtank . 54 Workman65 . 56 Index 58 scuba-package The Scuba Package Description This is a summary of the features of scuba, a package in R that performs theoretical calculations about scuba diving — dive profiles, decompression models, gas toxicity and so on. scuba-package 3 Details scuba is a package for performing calculations in the theory of scuba diving. -
Decompression-Theory.Pdf
Decompression Theory An Explanation of Professor A.A. Buehlmann's ZH-L16 Algorithm by Paul Chapman The following is a summary of the decompression algorithm described by Dr A.A. Buehlmann in the fourth Note to new divers and potential edition of his book Tauchmedizin ( new divers: diving medicine ) published in 1995 ( only in German. ) The book contains This information is presented for a considerable amount of other general interest. Don't be scared off information and is published by by what you see here - you don't Springer-Verlag ISBN 3-540-58970 need to learn any of this to become a 8. Rumor has it that at the time of safe and competent scuba diver. You writing ( November 1999 ) an English will however need to understand translation is being prepared for dive planning. publishing, so hopefully, in due course, this document will become redundant. The algorithm is simply a "recipe" for modeling the behavior of inert gases, which diffuse in and out of our body tissues when breathed under varying pressures. The intention is that if the recipe models the actual processes in our bodies accurately enough, it can be used to plan dives ( and other pressure exposures ) with a view to avoiding decompression sickness. It is important to realize that the model is entirely arbitrary in the sense that it in no way represents the actual physical processes which are taking place, it simply attempt to model the real-life results mathematically. This article is intended mainly as a description of the algorithm, not as a complete description of decompression physiology and therefore mentions only physiology principles relevant to the algorithm.