Strong Plasmon–Exciton Coupling in Ag Nanoparticle—Conjugated Polymer Core-Shell Hybrid Nanostructures
Total Page:16
File Type:pdf, Size:1020Kb
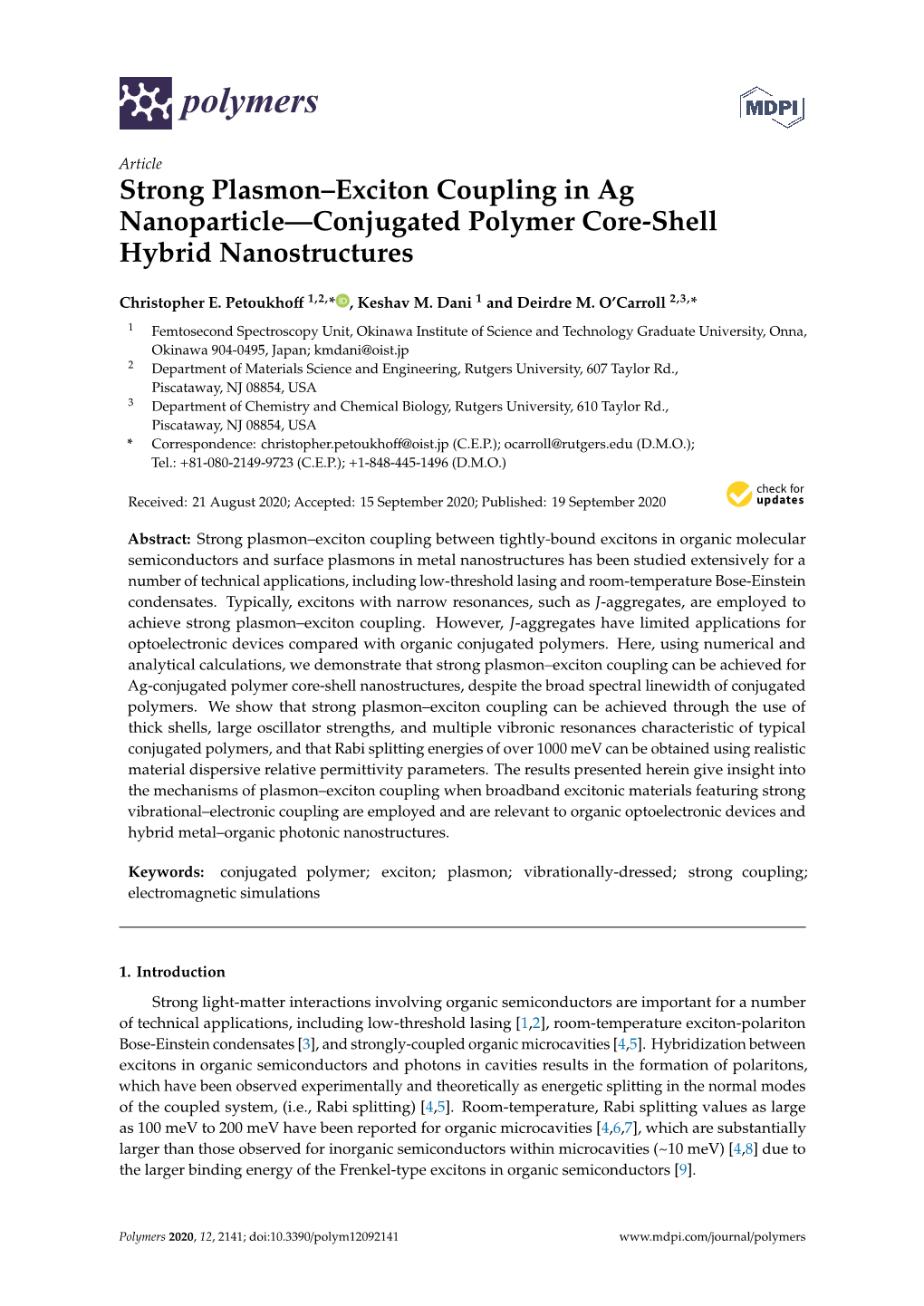
Load more
Recommended publications
-
2 a Primer on Polymer Colloids: Structure, Synthesis and Colloidal Stability
A. Al Shboul, F. Pierre, and J. P. Claverie 2 A primer on polymer colloids: structure, synthesis and colloidal stability 2.1 Introduction A colloid is a dispersion of very fine objects in a fluid [1]. These objects can be solids, liquids or gas, and the corresponding colloidal dispersion is then referred to as sus- pension, emulsion or foam. Colloids possess unique characteristics. For example, as their size is smaller than the wavelength of light, they scatter light. They also offer a large interfacial surface area, meaning that interfacial phenomena are of paramount importance in these dispersions. The weight of each dispersed particle being small, gravity and buoyancy forces are not sufficient to counteract the thermal random mo- tion of the particle, named Brownian motion (in tribute to the 19th century botanist Robert Brown who first characterized it). The particles do not remain in a dispersed state indefinitely: they will sooner or later aggregate (phase separation). Thus, thecol- loidal state is in general metastable and colloidal stability is one of the key features to take into account when working with colloids. Among all colloids, the polymer colloid family is one of the most widely inves- tigated [2]. Polymer colloids are used for a large number of applications, ranging from coatings, adhesives, inks, impact modifiers, drug-delivery vehicles, etc. The particles range in size from about 10 nm to 1 000 nm (1 μm) in diameter. They are usually spherical, but numerous other shapes have been observed. Polymer colloids are not uncommon in nature. For example, natural rubber latex, the secretion of the Hevea brasiliensis tree, is in fact a dispersion of polyisoprene nanoparticles in wa- ter. -
Entropy Driven Phase Transition in Polymer Gels: Mean Field Theory
entropy Article Entropy Driven Phase Transition in Polymer Gels: Mean Field Theory Miron Kaufman Physics Department, Cleveland State University, Cleveland, OH 44115, USA; [email protected] Received: 16 April 2018; Accepted: 27 June 2018; Published: 30 June 2018 Abstract: We present a mean field model of a gel consisting of P polymers, each of length L and Nz polyfunctional monomers. Each polyfunctional monomer forms z covalent bonds with the 2P bifunctional monomers at the ends of the linear polymers. We find that the entropy dependence on the number of polyfunctional monomers exhibits an abrupt change at Nz = 2P/z due to the saturation of possible crosslinks. This non-analytical dependence of entropy on the number of polyfunctionals generates a first-order phase transition between two gel phases: one poor and the other rich in poly-functional molecules. Keywords: crosslinking entropy; saturation; discontinuous phase transition 1. Motivation A polymer gel like polyacrylamide changes the volumes by a large factor of ~1000 when a small quantity of solvent like acetone is added to the solution or when the temperature is varied slightly. Central to the understanding of this phase transition are the covalent crosslinks [1–5] between the linear chains. Polymer chains, such as in hydroxypropylcellulose (HPC) immersed in a water solution, form hydrogen bonds with the water. As the temperature, the pH, or some other external condition is varied, there is a change in the strength of the hydrogen bonds that results in the formation or destruction of aggregates of crosslinked polymer chains. Light scattering experiments [6,7] are used to study the influence of the amount of crosslinkers on the properties of the gel phases. -
3.4 V-A Coupling of Leptons and Quarks 3.5 CKM Matrix to Describe the Quark Mixing
Advanced Particle Physics: VI. Probing the weak interaction 3.4 V-A coupling of leptons and quarks Reminder u γ µ (1− γ 5 )u = u γ µu L = (u L + u R )γ µu L = u Lγ µu L l ν l ν l l ν l ν In V-A theory the weak interaction couples left-handed lepton/quark currents (right-handed anti-lepton/quark currents) with an universal coupling strength: 2 GF gw = 2 2 8MW Weak transition appear only inside weak-isospin doublets: Not equal to the mass eigenstate Lepton currents: Quark currents: ⎛ν ⎞ ⎛ u ⎞ 1. ⎜ e ⎟ j µ = u γ µ (1− γ 5 )u 1. ⎜ ⎟ j µ = u γ µ (1− γ 5 )u ⎜ − ⎟ eν e ν ⎜ ⎟ du d u ⎝e ⎠ ⎝d′⎠ ⎛ν ⎞ ⎛ c ⎞ 5 2. ⎜ µ ⎟ j µ = u γ µ (1− γ 5 )u 2. ⎜ ⎟ j µ = u γ µ (1− γ )u ⎜ − ⎟ µν µ ν ⎜ ⎟ sc s c ⎝ µ ⎠ ⎝s′⎠ ⎛ν ⎞ ⎛ t ⎞ µ µ 5 3. ⎜ τ ⎟ j µ = u γ µ (1− γ 5 )u 3. ⎜ ⎟ j = u γ (1− γ )u ⎜ − ⎟ τν τ ν ⎜ ⎟ bt b t ⎝τ ⎠ ⎝b′⎠ 3.5 CKM matrix to describe the quark mixing One finds that the weak eigenstates of the down type quarks entering the weak isospin doublets are not equal to the their mass/flavor eigenstates: ⎛d′⎞ ⎛V V V ⎞ ⎛d ⎞ d V u ⎜ ⎟ ⎜ ud us ub ⎟ ⎜ ⎟ ud ⎜ s′⎟ = ⎜Vcd Vcs Vcb ⎟ ⋅ ⎜ s ⎟ ⎜ ⎟ ⎜ ⎟ ⎜ ⎟ W ⎝b′⎠ ⎝Vtd Vts Vtb ⎠ ⎝b⎠ Cabibbo-Kobayashi-Maskawa mixing matrix The quark mixing is the origin of the flavor number violation of the weak interaction. -
Soft Condensed Matter Physics Programme Course 6 Credits Mjuka Material TFYA37 Valid From: 2018 Spring Semester
1(9) Soft Condensed Matter Physics Programme course 6 credits Mjuka material TFYA37 Valid from: 2018 Spring semester Determined by Board of Studies for Electrical Engineering, Physics and Mathematics Date determined LINKÖPING UNIVERSITY FACULTY OF SCIENCE AND ENGINEERING LINKÖPING UNIVERSITY SOFT CONDENSED MATTER PHYSICS FACULTY OF SCIENCE AND ENGINEERING 2(9) Main field of study Applied Physics, Physics Course level Second cycle Advancement level A1X Course offered for Biomedical Engineering, M Sc in Engineering Engineering Biology, M Sc in Engineering Entry requirements Note: Admission requirements for non-programme students usually also include admission requirements for the programme and threshold requirements for progression within the programme, or corresponding. Prerequisites Mandatory courses in mathematics and physics for the Y-program or equal. Intended learning outcomes The course will ive the student knowledge of the statistical physics of polymers, the chemical, geometrical and electronic structure of polymers as well as the structure, dynamics and processing of polymer solids. We will discuss condensed matter in the form of colloids, amphiphiles, liquid crystals, molecular crystals and biological matter. After the course, the student should be able to describe the geometry of polymer chains and their dynamics, and the mathematical description of these phenomena utilize thermodynamical analysis of phase transitions in polymers and polymer blends LINKÖPING UNIVERSITY SOFT CONDENSED MATTER PHYSICS FACULTY OF SCIENCE AND ENGINEERING 3(9) describe micro and nanostructure of polymer solutions and polymer blends describe amphiphile materials, colloids, foams and gels, liquid crystals Course content Polymers: terminology, chemical structures and polymerization, solid state structures, polymers in solution, colligative properties. Statistical physics of polymer chains: random coils, entropy measures, rubber physics. -
UNIVERSITY of CALIFORNIA, SAN DIEGO Exciton Transport
UNIVERSITY OF CALIFORNIA, SAN DIEGO Exciton Transport Phenomena in GaAs Coupled Quantum Wells A dissertation submitted in partial satisfaction of the requirements for the degree Doctor of Philosophy in Physics by Jason R. Leonard Committee in charge: Professor Leonid V. Butov, Chair Professor John M. Goodkind Professor Shayan Mookherjea Professor Charles W. Tu Professor Congjun Wu 2016 Copyright Jason R. Leonard, 2016 All rights reserved. The dissertation of Jason R. Leonard is approved, and it is acceptable in quality and form for publication on microfilm and electronically: Chair University of California, San Diego 2016 iii TABLE OF CONTENTS Signature Page . iii Table of Contents . iv List of Figures . vi Acknowledgements . viii Vita........................................ x Abstract of the Dissertation . xii Chapter 1 Introduction . 1 1.1 Semiconductor introduction . 2 1.1.1 Bulk GaAs . 3 1.1.2 Single Quantum Well . 3 1.1.3 Coupled-Quantum Wells . 5 1.2 Transport Physics . 7 1.3 Spin Physics . 8 1.3.1 D'yakanov and Perel' spin relaxation . 10 1.3.2 Dresselhaus Interaction . 10 1.3.3 Electron-Hole Exchange Interaction . 11 1.4 Dissertation Overview . 11 Chapter 2 Controlled exciton transport via a ramp . 13 2.1 Introduction . 13 2.2 Experimental Methods . 14 2.3 Qualitative Results . 14 2.4 Quantitative Results . 16 2.5 Theoretical Model . 17 2.6 Summary . 19 2.7 Acknowledgments . 19 Chapter 3 Controlled exciton transport via an optically controlled exciton transistor . 22 3.1 Introduction . 22 3.2 Realization . 22 3.3 Experimental Methods . 23 3.4 Results . 25 3.5 Theoretical Model . -
Shape Memory and Actuation Behavior of Semicrystalline Polymer Networks
Dipl.-Phys. Martin Bothe Shape Memory and Actuation Behavior of Semicrystalline Polymer Networks BAM-Dissertationsreihe • Band 121 Berlin 2014 Die vorliegende Arbeit entstand an der BAM Bundesanstalt für Materialforschung und -prüfung. Impressum Shape Memory and Actuation Behavior of Semicrystalline Polymer Networks 2014 Herausgeber: BAM Bundesanstalt für Materialforschung und -prüfung Unter den Eichen 87 12205 Berlin Telefon: +49 30 8104-0 Telefax: +49 30 8112029 E-Mail: [email protected] Internet: www.bam.de Copyright © 2014 by BAM Bundesanstalt für Materialforschung und -prüfung Layout: BAM-Referat Z.8 ISSN 1613-4249 ISBN 978-3-9816668-1-6 Shape Memory and Actuation Behavior of Semicrystalline Polymer Networks vorgelegt von Dipl.-Phys. Martin Bothe aus Tubingen¨ von der Fakult¨at II – Mathematik und Naturwissenschaften der Technischen Universit¨at Berlin zur Erlangung des akademischen Grades Doktor der Naturwissenschaften – Dr. rer. nat. – genehmigte Dissertation Promotionsausschuss: Vorsitzender: Prof. Dr.-Ing. Matthias Bickermann Gutachter: Prof. Dr. rer. nat. Michael Gradzielski Gutachter: Prof. Dr. rer. nat. Michael Maskos Tag der wissenschaftlichen Aussprache: 16.07.2014 Berlin 2014 D 83 Für meine Familie Abstract Shape memory polymers (SMPs) can change their shape on application of a suitable stimulus. To enable such behavior, a ‘programming’ procedure fixes a deformation, yielding a stable tem- porary shape. In thermoresponsive SMPs, subsequent heating triggers entropy-elastic recovery of the initial shape. An additional shape change on cooling, i.e. thermoreversible two-way actuation, can be stimulated by a crystallization phenomenon. In this thesis, cyclic thermomechanical measurements systematically determined (1) the shape memory and (2) the actuation behavior under constant load as well as under stress-free condi- tions. -
Strong Coupling and Quantum Molecular Plasmonics
Strong coupling and molecular plasmonics Javier Aizpurua http://cfm.ehu.es/nanophotonics Center for Materials Physics, CSIC-UPV/EHU and Donostia International Physics Center - DIPC Donostia-San Sebastián, Basque Country AMOLF Nanophotonics School June 17-21, 2019, Science Park, Amsterdam, The Netherlands Organic molecules & light Excited molecule (=exciton on molecule) Photon Photon Adding mirrors to this interaction The photon can come back! Optical cavities to enhance light-matter interaction Optical mirrors Dielectric resonator Veff Q ∼ 1/κ Photonic crystals Plasmonic cavity Veff Q∼1/κ Coupling of plasmons and molecular excitations Plasmon-Exciton Coupling Plasmon-Vibration Coupling Absorption, Scattering, IR Absorption, Fluorescence Veff Raman Scattering Extreme plasmonic cavities Top-down Bottom-up STM ultra high-vacuum Wet Chemistry Low temperature Self-assembled monolayers (Hefei, China) (Cambridge, UK) “More interacting system” One cannot distinguish whether we have a photon or an excited molecule. The eigenstates are “hybrid” states, part molecule and part photon: “polaritons” Strong coupling! Experiments: organic molecules Organic molecules: Microcavity: D. G. Lidzey et al., Nature 395, 53 (1998) • Large dipole moments • High densities collective enhancement • Rabi splitting can be >1 eV (~30% of transition energy) • Room temperature! Surface plasmons: J. Bellessa et al., Single molecule with gap plasmon: Phys. Rev. Lett. 93, 036404 (2004) Chikkaraddy et al., Nature 535, 127 (2016) from lecture by V. Shalaev Plasmonic cavities -
Polymer Exemption Guidance Manual POLYMER EXEMPTION GUIDANCE MANUAL
United States Office of Pollution EPA 744-B-97-001 Environmental Protection Prevention and Toxics June 1997 Agency (7406) Polymer Exemption Guidance Manual POLYMER EXEMPTION GUIDANCE MANUAL 5/22/97 A technical manual to accompany, but not supersede the "Premanufacture Notification Exemptions; Revisions of Exemptions for Polymers; Final Rule" found at 40 CFR Part 723, (60) FR 16316-16336, published Wednesday, March 29, 1995 Environmental Protection Agency Office of Pollution Prevention and Toxics 401 M St., SW., Washington, DC 20460-0001 Copies of this document are available through the TSCA Assistance Information Service at (202) 554-1404 or by faxing requests to (202) 554-5603. TABLE OF CONTENTS LIST OF EQUATIONS............................ ii LIST OF FIGURES............................. ii LIST OF TABLES ............................. ii 1. INTRODUCTION ............................ 1 2. HISTORY............................... 2 3. DEFINITIONS............................. 3 4. ELIGIBILITY REQUIREMENTS ...................... 4 4.1. MEETING THE DEFINITION OF A POLYMER AT 40 CFR §723.250(b)... 5 4.2. SUBSTANCES EXCLUDED FROM THE EXEMPTION AT 40 CFR §723.250(d) . 7 4.2.1. EXCLUSIONS FOR CATIONIC AND POTENTIALLY CATIONIC POLYMERS ....................... 8 4.2.1.1. CATIONIC POLYMERS NOT EXCLUDED FROM EXEMPTION 8 4.2.2. EXCLUSIONS FOR ELEMENTAL CRITERIA........... 9 4.2.3. EXCLUSIONS FOR DEGRADABLE OR UNSTABLE POLYMERS .... 9 4.2.4. EXCLUSIONS BY REACTANTS................ 9 4.2.5. EXCLUSIONS FOR WATER-ABSORBING POLYMERS........ 10 4.3. CATEGORIES WHICH ARE NO LONGER EXCLUDED FROM EXEMPTION .... 10 4.4. MEETING EXEMPTION CRITERIA AT 40 CFR §723.250(e) ....... 10 4.4.1. THE (e)(1) EXEMPTION CRITERIA............. 10 4.4.1.1. LOW-CONCERN FUNCTIONAL GROUPS AND THE (e)(1) EXEMPTION................. -
Two-Plasmon Spontaneous Emission from a Nonlocal Epsilon-Near-Zero Material ✉ ✉ Futai Hu1, Liu Li1, Yuan Liu1, Yuan Meng 1, Mali Gong1,2 & Yuanmu Yang1
ARTICLE https://doi.org/10.1038/s42005-021-00586-4 OPEN Two-plasmon spontaneous emission from a nonlocal epsilon-near-zero material ✉ ✉ Futai Hu1, Liu Li1, Yuan Liu1, Yuan Meng 1, Mali Gong1,2 & Yuanmu Yang1 Plasmonic cavities can provide deep subwavelength light confinement, opening up new avenues for enhancing the spontaneous emission process towards both classical and quantum optical applications. Conventionally, light cannot be directly emitted from the plasmonic metal itself. Here, we explore the large field confinement and slow-light effect near the epsilon-near-zero (ENZ) frequency of the light-emitting material itself, to greatly enhance the “forbidden” two-plasmon spontaneous emission (2PSE) process. Using degenerately- 1234567890():,; doped InSb as the plasmonic material and emitter simultaneously, we theoretically show that the 2PSE lifetime can be reduced from tens of milliseconds to several nanoseconds, com- parable to the one-photon emission rate. Furthermore, we show that the optical nonlocality may largely govern the optical response of the ultrathin ENZ film. Efficient 2PSE from a doped semiconductor film may provide a pathway towards on-chip entangled light sources, with an emission wavelength and bandwidth widely tunable in the mid-infrared. 1 State Key Laboratory of Precision Measurement Technology and Instruments, Department of Precision Instrument, Tsinghua University, Beijing, China. ✉ 2 State Key Laboratory of Tribology, Department of Mechanical Engineering, Tsinghua University, Beijing, China. email: [email protected]; [email protected] COMMUNICATIONS PHYSICS | (2021) 4:84 | https://doi.org/10.1038/s42005-021-00586-4 | www.nature.com/commsphys 1 ARTICLE COMMUNICATIONS PHYSICS | https://doi.org/10.1038/s42005-021-00586-4 lasmonics is a burgeoning field of research that exploits the correction of TPE near graphene using the zero-temperature Plight-matter interaction in metallic nanostructures1,2. -
The Electron-Phonon Coupling Constant
The electron-phonon coupling constant Philip B. Allen Department of Physics and Astronomy, State University of New York, Stony Brook, NY 11794-3800 March 17, 2000 Tables of values of the electron-phonon coupling constants and are given for selected tr elements and comp ounds. A brief summary of the theory is also given. I. THEORETICAL INTRODUCTION Grimvall [1] has written a review of electron-phonon e ects in metals. Prominent among these e ects is sup ercon- ductivity. The BCS theory gives a relation T exp1=N 0V for the sup erconducting transition temp erature c D T in terms of the Debye temp erature .Values of T are tabulated in various places, the most complete prior to c D c the high T era b eing ref. [2]. The electron-electron interaction V consists of the attractive electron-phonon-induced c interaction minus the repulsive Coulombinteraction. The notation is used = N 0V 1 eph and the Coulomb repulsion N 0V is called , so that N 0V = , where is a \renormalized" Coulomb c repulsion, reduced in value from to =[1 + ln! =! ]. This suppression of the Coulomb repulsion is a result P D of the fact that the electron-phonon attraction is retarded in time by an amountt 1=! whereas the repulsive D screened Coulombinteraction is retarded byamuch smaller time, t 1=! where ! is the electronic plasma P P frequency. Therefore, is b ounded ab oveby1= ln! =! which for conventional metals should b e 0:2. P D Values of are known to range from 0:10 to 2:0. -
How the Electron-Phonon Coupling Mechanism Work in Metal Superconductor
How the electron-phonon coupling mechanism work in metal superconductor Qiankai Yao1,2 1College of Science, Henan University of Technology, Zhengzhou450001, China 2School of physics and Engineering, Zhengzhou University, Zhengzhou450001, China Abstract Superconductivity in some metals at low temperature is known to arise from an electron-phonon coupling mechanism. Such the mechanism enables an effective attraction to bind two mobile electrons together, and even form a kind of pairing system(called Cooper pair) to be physically responsible for superconductivity. But, is it possible by an analogy with the electrodynamics to describe the electron-phonon coupling as a resistivity-dependent attraction? Actually so, it will help us to explore a more operational quantum model for the formation of Cooper pair. In particularly, by the calculation of quantum state of Cooper pair, the explored model can provide a more explicit explanation for the fundamental properties of metal superconductor, and answer: 1) How the transition temperature of metal superconductor is determined? 2) Which metals can realize the superconducting transition at low temperature? PACS numbers: 74.20.Fg; 74.20.-z; 74.25.-q; 74.20.De ne is the mobile electron density, η the damping coefficient 1. Introduction that is determined by the collision time τ . In the BCS theory[1], superconductivity is attributed to a In metal environment, mobile electrons are usually phonon-mediated attraction between mobile electrons near modeled to be a kind of classical particles like gas molecules, Fermi surface(called Fermi electrons). The attraction is each of which performs a Brown-like motion and satisfies the sometimes referred to as a residual Coulomb interaction[2] that Langevin equation can glue Cooper pair together to cause superconductivity. -
Chapter 09584
Author's personal copy Excitons in Magnetic Fields Kankan Cong, G Timothy Noe II, and Junichiro Kono, Rice University, Houston, TX, United States r 2018 Elsevier Ltd. All rights reserved. Introduction When a photon of energy greater than the band gap is absorbed by a semiconductor, a negatively charged electron is excited from the valence band into the conduction band, leaving behind a positively charged hole. The electron can be attracted to the hole via the Coulomb interaction, lowering the energy of the electron-hole (e-h) pair by a characteristic binding energy, Eb. The bound e-h pair is referred to as an exciton, and it is analogous to the hydrogen atom, but with a larger Bohr radius and a smaller binding energy, ranging from 1 to 100 meV, due to the small reduced mass of the exciton and screening of the Coulomb interaction by the dielectric environment. Like the hydrogen atom, there exists a series of excitonic bound states, which modify the near-band-edge optical response of semiconductors, especially when the binding energy is greater than the thermal energy and any relevant scattering rates. When the e-h pair has an energy greater than the binding energy, the electron and hole are no longer bound to one another (ionized), although they are still correlated. The nature of the optical transitions for both excitons and unbound e-h pairs depends on the dimensionality of the e-h system. Furthermore, an exciton is a composite boson having integer spin that obeys Bose-Einstein statistics rather than fermions that obey Fermi-Dirac statistics as in the case of either the electrons or holes by themselves.