Considering Ecological Processes in Environmental Impact Assessments
Total Page:16
File Type:pdf, Size:1020Kb
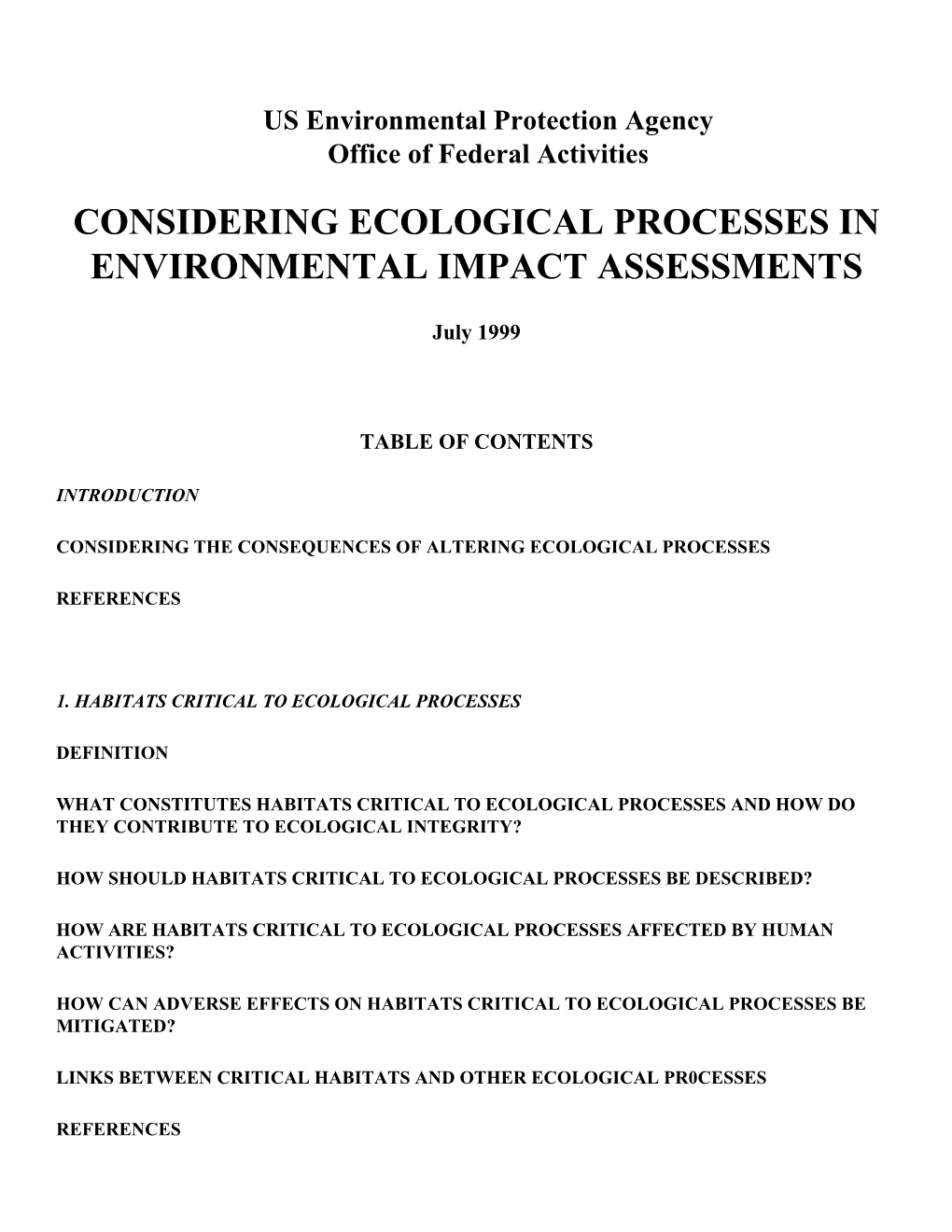
Load more
Recommended publications
-
Ecological Principles and Function of Natural Ecosystems by Professor Michel RICARD
Intensive Programme on Education for sustainable development in Protected Areas Amfissa, Greece, July 2014 ------------------------------------------------------------------------ Ecological principles and function of natural ecosystems By Professor Michel RICARD Summary 1. Hierarchy of living world 2. What is Ecology 3. The Biosphere - Lithosphere - Hydrosphere - Atmosphere 4. What is an ecosystem - Ecozone - Biome - Ecosystem - Ecological community - Habitat/biotope - Ecotone - Niche 5. Biological classification 6. Ecosystem processes - Radiation: heat, temperature and light - Primary production - Secondary production - Food web and trophic levels - Trophic cascade and ecology flow 7. Population ecology and population dynamics 8. Disturbance and resilience - Human impacts on resilience 9. Nutrient cycle, decomposition and mineralization - Nutrient cycle - Decomposition 10. Ecological amplitude 11. Ecology, environmental influences, biological interactions 12. Biodiversity 13. Environmental degradation - Water resources degradation - Climate change - Nutrient pollution - Eutrophication - Other examples of environmental degradation M. Ricard: Summer courses, Amfissa July 2014 1 1. Hierarchy of living world The larger objective of ecology is to understand the nature of environmental influences on individual organisms, populations, communities and ultimately at the level of the biosphere. If ecologists can achieve an understanding of these relationships, they will be well placed to contribute to the development of systems by which humans -
Chapter 3. Nutrient Cycling by Fungi in Wet Tropical Forests
Chapter 3 Nutrient cycling by fungi in wet tropical forests D. Jean Lodge U.S.D.A. Forest Service, Forest Products Laboratory, Center for Forest Mycology Reseach, PO Box B, Palmer, Pueto Rico 00721, U.S.A. Introduction Fungi are primarily responsible for the recycling of mineral nutrients through decomposition of organic matter (Swift, Heal & Anderson, 1979) and the uptake and transfer of these nutrients into plants via mycorrizal fungi (Janos, 1983). In addition, fungi and other soil microorganisms serve alternately as sources and sinks of labile nutrients that are necessary for plant growth (Marumoto, Anderson & Domsch 1983 Yang & Insam 1991). Thus, fungal and microbial biomass can control significant fractions of the labile nutrient pools in some humid and wet tropical forests (Marumoto et al., 1982; Lodge, 1985; Yang & Insam, 1991), and regulate the availability of nutrients that may limit plant growth (Jordan 1985; Hilton, 1987; Singh et al., 1989 Lee, Han & Jordan 1990 Behera Pati & Basu, 1991; Yang & Insam, 1991). The term biomass normally refers only to living organisms, but it is used more broadly in this chapter to refer to dead as well as living microorganisms as both contain nutrients. Although humid tropical forests often have large stature and an abundance of vegetation, their growth and productivity is frequently limited by the availability of mineral nutrients. A diversity of soils occurs in the wet tropics so it is difficult to generalise about nutrient limitation. However, the availability of phosphorus to higher plants is generally limited because phosphorus combines with aluminium and iron oxides in the highly weathered soils to form insoluble complexes (Sanchez 1976). -
The Importance of Soil Food Web for Healthy Environment and Sustainable Development
International Journal of Applied Research 2015; 1(3): 15-20 ISSN Print: 2394-7500 ISSN Online: 2394-5869 Impact Factor: 3.4 The importance of soil food web for healthy IJAR 2015; 1(3): 15-20 www.allresearchjournal.com environment and sustainable development Received: 18-01-2015 Accepted: 08-02-2015 Rajeev Ranjan, M. Divya and M. Bavitha Rajeev Ranjan Abstract PG Research Scholar, Soil food web is a natural network of consumer resource interactions among different functional Dept. of Aquaculture, groups of soil organisms which are occur in the soil ecosystem. Soil is a complex, unconsolidated Fisheries College & Research mixture of inorganic, organic, and living material that is found on the immediate surface of the earth Institute, TNFU, that supports many important functions for plants, animals, and humans. The soil food web is very Thoothukudi-8, dynamic, complex and interchanging depending on its ecosystem. Nutrients in soil in their most basic Tamil Nadu, India. form come from fully decomposed organic matter which we call compost. Decomposition of organic matter is largely a biological process that occurs naturally. The organisms found in the soil food web M. Divya PG Research Scholar, carry out a large amount of microbial processes such as decomposition, mineralization, Dept. of Aquatic environment immobilization, respiration, and fixation along with many others. Without soil food web, plants management, would not obtain the nutrients which are necessary for growth. Nutrient exchanges between organic Fisheries College & Research matter, water and soil are essential to soil fertility and need to be maintained for sustainable Institute, TNFU, Thoothukudi- production. A diverse and complete soil food web where soils are well structured and fertile through 8, T.N. -
Determination of the Denitrification Capacity of Unconsolidated Rock
Determination of the denitrification capacity of unconsolidated rock aquifers using 15N tracer experiments at groundwater monitoring wells - development of a new method to assess actual and future denitrification in aquifers Dissertation zur Erlangung des Doktorgrades der Fakultät für Forstwissenschaften und Waldökologie der Georg-August-Universität Göttingen vorgelegt von Diplom Geologe Wolfram Eschenbach geboren in Burg Göttingen, 2014 1. Gutachter: Prof. Dr. Heinz Flessa 2. Gutachter: Prof. Dr. Jürgen Böttcher Tag der mündlichen Prüfung: 28.01.2014 "Ich bin mir jedenfalls bewusst, dass ich keine Weisheit besitze, weder groß noch klein." Sokrates Table of contents Figures ...................................................................................................................................... V Tables ....................................................................................................................................... VI Danksagung ........................................................................................................................... VII Abstract ................................................................................................................................... IX Kurzfassung ............................................................................................................................ XI Preface and Outline ............................................................................................................. XIII 1 General Introduction ......................................................................................................... -
Impacts from Harvests and Prescribed Burns to the Nutrient Cycle of Pine Plantations
Technical Resource Bulletin January 2000 TRB-003 Impacts from Harvests and Prescribed Burns to the Nutrient Cycle of Pine Plantations Bill Pickens, Conifer Silviculturist Introduction Forest trees require 16 basic nutrient elements to grow Controlled burning is a widely accepted forestry and maintain health. Three of these elements, oxygen, practice in the Southeast and a common site carbon and hydrogen are derived from water and air. preparation practice at Bladen Lakes State Forest in The rest are supplied by the soil. The "Big Three" North Carolina. A prescribed burn is used to eliminate macronutrients, Nitrogen (N), phosphorus (P), and residual logging debris, reduce vegetative competition, potassium (K), comprise over 67% of the nutrients and improve accessibility for tree planting. Ultimately, found in plant tissue and are used in large quantities by the goal is to enhance the success of seedling forest trees (Landis 2003). Calcium, magnesium, and establishment. sulfur are used in smaller quantities but are just as important to plant health and vigor. The seven micro- This paper reviews the impact harvest and prescribe nutrients, boron, chlorine, copper, iron, manganese, burning for site preparation on the nutrient cycle of molybdenum, and zinc, while used in very small forest trees. amounts are essential to plant metabolism. Harvest Impacts The nutrient cycle is a process where the mineral Harvesting removes nutrients by removing biomass. A nutrients are absorbed by the tree and then returned to majority of the nutrients located in the tree biomass are the soil to be used again. The mineral nutrients are concentrated in the foliage, branches, and root system. -
Nutrient Cycling Proceedings I
Livestock and Sustainable Nutrient Cycling in Mixed Farming Systems of sub-Saharan Africa Volume I: Conference Summary Proceedings of an International Conference International Livestock Centre for Africa (ILCA) Addis Ababa, Ethiopia 22–26 November 1993 Edited by J. M. Powell, S. Fernández-Rivera, T.O. Williams and C. Renard CORE Metadata, citation and similar papers at core.ac.uk Provided by CGSpace ISBN 92–9053–291–2 Correct citation: Powell J M, Fernández-Rivera S, Williams T O and Renard C. 1994. Livestock and Sustainable Nutrient Cycling in Mixed Farming Systems of sub-Saharan Africa. Volume I: Conference Summary. Proceedings of an International Conference held in Addis Ababa, Ethiopia, 22–26 November 1993. ILCA (International Livestock Centre for Africa), Addis Ababa, Ethiopia. 55 pp. Table of Contents Page Acknowledgements Foreword Programme Summary of Plenary Sessions Opening Session Interactions between animals and plants Interactions between animals and soils Interactions between plants and soils Nutrient cycling in mixed farming systems Modelling nutrient cycles Closing Session Summary of Focus Group Sessions Introduction Animal–plant interactions Animal–soil interactions Socio-economic dimensions in nutrient cycling Appendix I: Abstracts Appendix II: List of Participants Livestock and sustainable nutrient cycling iii Acknowledgements We gratefully acknowledge the contributions from the following sponsors: International Crops Research Institute for the Semi-Arid Tropics (ICRISAT) International Centre for Research in Agroforestry -
Nutrient Cycling (Chapters 16-18) Lab Today – Finish Inventory Work At
Lubrecht Forest, Montana NREM 301 Forest Ecology & Soils Day 23 – Nov 10, 2009 Nutrient Cycling (Chapters 16-18) Lab today – Finish Inventory work at Rest Area Site Quiz on Thursday Take a plot every 50 ft Teams 1- 4 Azimuth Teams 5-8Azimuth Plot 1m2 o o 0 or 360 or N 330o Identify by: 1. Warm-season grasses 2. Cool-season grasses 3. Forbs 4. Woody species 5. Litter 6. Bare ground 7. Standing dead woody material For Each of the above use an 8- point scale (0, Trace, 3%, 16%, 38%, 63%, 85%, 96%) Total can be more than 100% Set meter stick vertical in center of the plot – at 4 m from the stick and at 1 m above the ground write down the decimeter on stick that is 50% covered Also record trees & shrubs that cross the tape – note distance measure where they cross – keep track of total distance % classes for the following cover types: 0, Trace, 3, 16, 38, 63, 85, 96 Team Number in m2 plots (can exceed 100% total because of multiple canopies) Total Robel Reading Plot Distance Warm‐ Cool‐season Woody Standing 4 m away 1 m Numberfrom start season Grass Grass Forbs Species Litter Bare Ground dead wood above ground 1 2 3 4 5 6 7 8 9 10 11 12 13 14 15 16 17 18 19 20 21 22 Team Number Tree/shrub Inventory ‐ note whenever a tree or shrub stem or branch crosses your transect ‐ list at least shrub or tree & if you can ID please do ‐ start in 2 left columns and down then move right Distance from Tree or shrub species Distance from Tree or shrub species Distance from Tree or shrub species beginning beginning beginning Group Activity Diagram the general nutrient cycle for an ecosystem Think of nutrients cycling in an ecosystem box a) Inputs (how do nutrients get into an ecosystem) b) Outputs (how are nutrients lost from the ecosystem) c) Internal Cycling (how do nutrients move around in the ecosystem Biochemical General Nutrient Cycle Geochemical Biogeochemical Page 525 Textbook Ability of fresh OM to be incorporated into the soil helps dictate rate of cycling Oi (L) Oe (F) Oa (H) Mineral soil Bogs 1. -
Cycle of a Nutrient 5TH GRADE THEME: EXPLORING the ECOLOGY of FOOD 55 MIN
Cycle of a Nutrient 5TH GRADE THEME: EXPLORING THE ECOLOGY OF FOOD 55 MIN. SPRING ESSENTIAL QUESTIONS Where do the nutrients in our food come from? • During Action Step 4, suggest that Where do the nutrients in our food waste go? the teacher support and encourage Why is composting food waste an important students as they craft their Year in the step in the nutrient cycle? Life of a Nutrient representations. LEARNING OBJECTIVES LESSON DESCRIPTION Students will be able to explain how the nutri- In this lesson, students learn about the nutrient ents that nourish us are derived from soil and air. cycle and demonstrate their understanding of the Students will be able to explain how our food nutrient cycle through a cartoon or narration. waste can go back into the nutrient cycle in the form of compost to replenish the soil. MATERIALS Objects that represent the nutrient cycle, such as an CONCEPTS apple, a decaying apple core, a small container of compost cycle decomposers rich garden soil or finished compost, and a small twig Handkerchief or tray decomposition environment nutrient Cycle of a Nutrient Cards (p. 581) Nutrient Cycle Poster (p. 582) Paper for each student Engaging the Classroom Teacher Markers and colored pencils • Prior to the lesson, ask the teacher about students’ familiarity with ele- ments and the process of decomposi- PREPARATION tion. > Photocopy Cycle of a Nutrient Cards, and • Ask the teacher whether they have cut them out; create sets for partners. Set established groups of 3–5 students aside all the cards with images of a worm or a who work well collaboratively. -
Prescott's Microbiology 5E
Prescott−Harley−Klein: VIII. Ecology and 28. Microorganism © The McGraw−Hill Microbiology, Fifth Edition Symbiosis Interactions and Microbial Companies, 2002 Ecology 28.2 Microbial Interactions 601 Gill Gill plume plume O O 2 2 HSHbO2 CO2 CO2 H2S H2S Circulatory Vestimentum system Bacteria Sulfide Sulfide O2 Trophosome oxidation ATP Coelom Trophosome cell NAD(P)H Bacteria Calvin CO2 Capillary cycle Reduced Tube carbon compounds Capillary Nutrients Translocation Endosymbiotic products bacteria Opisthosome O2 CO 2 Animal tissues H2S (a) (b) (c) (d) Figure 28.6 The Tube Worm—Bacterial Relationship. (a) A community of tube worms (Riftia pachyptila) at the Galápagos Rift hydrothermal vent site (depth 2,550 m). Each worm is more than a meter in length and has a 20 cm gill plume. (b, c) Schematic illustration of the anatomical and physiological organization of the tube worm. The animal is anchored inside its protective tube by the vestimentum. At its anterior end is a respiratory gill plume. Inside the trunk of the worm is a trophosome consisting primarily of endosymbiotic bacteria, associated cells, and blood vessels. At the posterior end of the animal is the opisthosome, which anchors the worm in its tube. (d) Oxygen, carbon dioxide, and hydrogen sulfide are absorbed through the gill plume and transported to the blood cells of the trophosome. Hydrogen sulfide is bound to the worm’s hemoglobin (HSHbO2) and carried to the endosymbiont bacteria. The bacteria oxidize the hydrogen sulfide and use some of the released energy to fix CO2 in the Calvin cycle. Some fraction of the reduced carbon compounds synthesized by the endosymbiont is translocated to the animal’s tissues. -
The Economic Value and Opportunities of Nutrient Cycling for Finland
The economic value and Sitra Studies opportunities of nutrient 104 cycling for Finland Maija Aho, Tiina Pursula, Mari Saario, Tea Miller, Anna Kumpulainen, Minna Päällysaho, Venla Kontiokari, Miikka Autio, Anna Hillgren, Laura Descombes, Gaia Consulting October 2015 © Sitra 2015 Sitra Studies 104 ISBN 978-951-563-944-8 (PDF) www.sitra.fi ISSN 1796-7112 (PDF) www.sitra.fi Enquiries about the publication: [email protected] Photos: Joanna Moorhouse Sitra Studies is a Publication Series which focuses on the conclusions and outcomes of Sitra’s future- oriented work. Foreword THE CIRCULAR ECONOMY HAS RECENTLY BECOME A KEY On the basis of these surveys, Sitra, the Baltic Sea Ac- ISSUE IN Finland. In many cases, the focus is on waste recycling, tion Group and Lappeenranta University of Technolo- despite the greater potential value to be found in sustainable gy propose three goals in boosting Finland's economic manufacturing, the reuse and remanufacturing of products and growth and the status of the Baltic Sea: materials, and maintenance. In a circular economy, materials • By 2020, 10% of manure from Finland will be pro- and value would circulate within society while, say, services and cessed into precision fertilisers. Finland will invest in intelligent solutions added value to products. The objective is developing nutrient recovery technologies. to reduce waste and create a system with zero loss of material. • By 2023, 100% of biowaste will be recovered and According to the Sitra and McKinsey study “The opportu- recycled. nities of a circular economy for Finland” (2015), conservative • By 2035, municipal wastewater treatment processes estimates put the value creation potential of Finland's circu- will be modified to recycle and recover nitrogen and lar economy at EUR 1.5–2.5 billion in five investigated sec- carbon in addition to phosphorus. -
Nutrient Cycles in Marine Ecosystems
Nutrient Cycles in Marine Ecosystems Chapter 4 Nitrogen, Carbon, Magnesium, Calcium, Phophorous Chapter 4 vocabulary 1. Nutrient 6. Assimilation 11.Leaching 2. Decomposer 7. Residence time 12.Marine snow 3. Reservoir 8. Sink 13.Dissociation 4. Run-off 9. Source 14.Diazotroph 5. Upwelling 10.Infiltration 15.Saprophytic Cycling of elements in the ecosystem Nutrient cycles move nutrients that are essential for life through the food chain through feeding. Nutrients: a chemical that provides what is needed for organisms to live and grow. When organisms die, decomposers break down the organic tissue and return it to inorganic forms. (inorganic forms could remain in ecosystem for millions of years before being synthesized into organic forms) Decomposer: bacteria and fungi that break down dead organic matter and release the nutrients back into the environment. The ocean is a reservoir for elements….. Reservoir: part of the abiotic phase of the nutrient cycle where nutrients can remain for long periods of time. Producers and microorganisms are able to fix inorganic molecules to organic substances. In this way… the abiotic part of the cycle can be moved into the biotic. (Fecal matter/dead organisms) Some are added to coral reefs and/or removed from the ocean altogether from harvesting. Molecules are added in through run-off and upwelling. Run-off: the flow of water from land caused by precipitation Upwelling: the movement of cold, nutrient rich water from deep in the ocean to the surface CONSUMERS BIOTIC PHASE PRIMARY DECOMPOSERS PRODUCERS ABIOTIC PHASE Nutrients found as inorganic ions and compounds in the atmosphere, dissolved in water, forming rocks and sediments Nutrient cycles Nutrients move from from the abiotic to the biotic when nutrients are absorbed and assimilated by producers Assimilation: the conversion of a nutrient into a usable form that can be incorporated into the tissues of an organism. -
Discovery of Anammox Bacteria in Terrestrial Ecosystems
University of Neuchâtel, Institute of Biology, Laboratory of Microbiology Discovery of anammox bacteria in terrestrial ecosystems Thèse présentée à la Faculté des Sciences Institut de Biologie Université de Neuchâtel Pour l'obtention du grade de DOCTEUR ÈS SCIENCES Par SYLVIA HUMBERT Acceptée sur proposition du jury: Prof. Michel Aragno, directeur de thèse Dr. Jakob Zopfi, co-directeur de thèse Prof. Pilar Junier, rapporteur Prof. Jean-Michel Gobat, rapporteur Dr. Patricia Bonin, rapporteur Soutenue le 21 décembre 2010 Université de Neuchâtel 2011 A tous ceux qui ont eu la patience d'attendre. À Stéphane, à mes parents et ma sœur. This research project was funded by the Swiss State Secretariat for Science and Education-COST Action 856 (Project SER No. C04.0269). It was carried out at the Laboratory of Microbiology of the University of Neuchâtel (LAMUN) from October 2005 to September 2010. Remerciements Je tiens à exprimer ma profonde reconnaissance à toutes les personnes qui ont contribué d'une manière ou d'une autre, à la réalisation de ce travail: Au Professeur Michel Aragno pour m'avoir transmis sa passion de la microbiologie et ses connaissances. Pour m'avoir permis de le remplacer quelques fois à ses cours, mais surtout pour m'avoir donné la chance de réaliser ma thèse dans son laboratoire. À mes deux superviseurs Jakob Zopfi et Sonia Tarnawski pour leur idées, leur savoir, leurs expériences et leurs conseils qu'ils ont su me donner tout au long de ces années de travail. Ils ont aussi su montrer beaucoup de patience pendant les cinq années d'encadrement.