Prokaryote Communities at Active Chimney and In-Situ Colonization
Total Page:16
File Type:pdf, Size:1020Kb
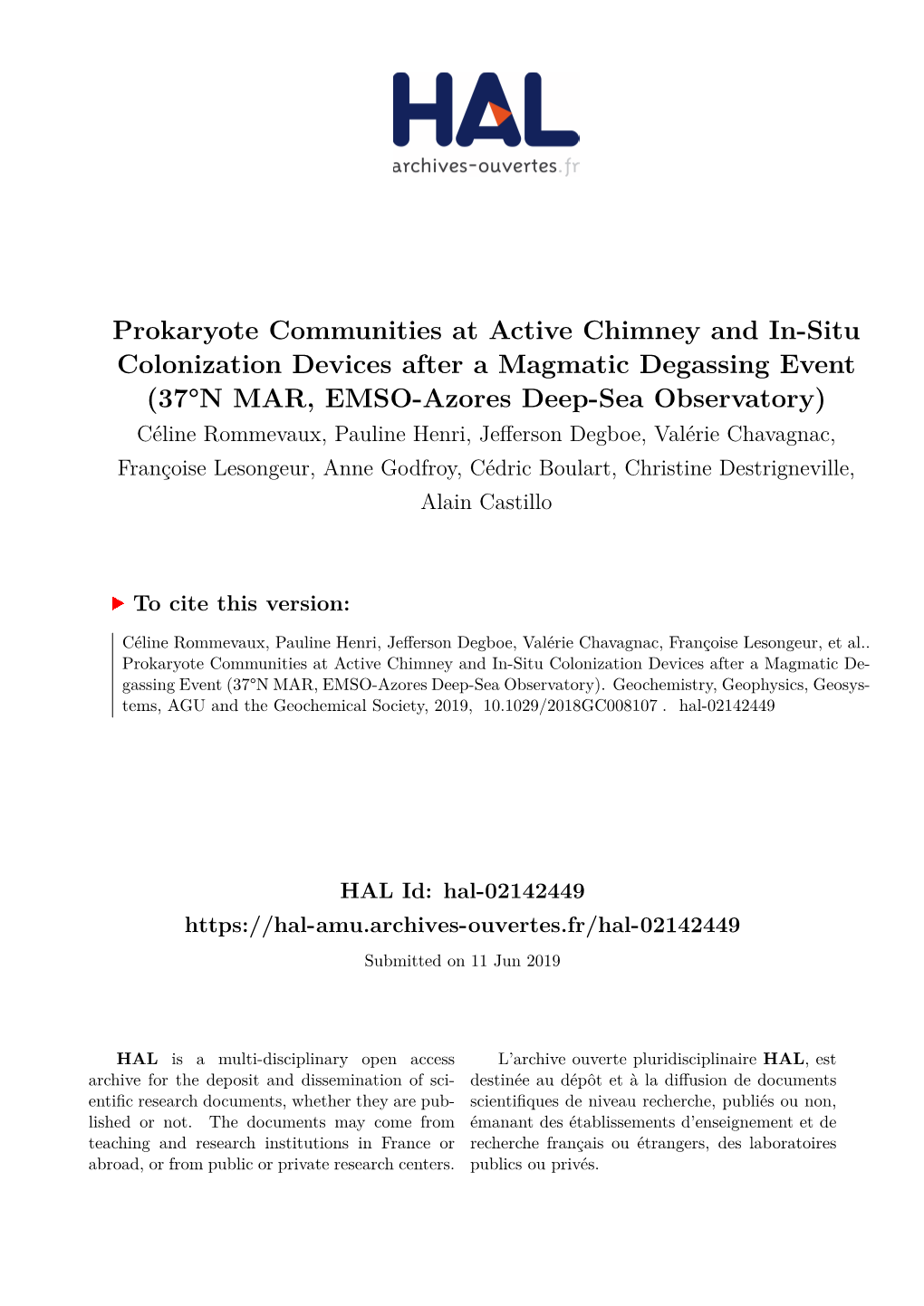
Load more
Recommended publications
-
Intra-Field Variation of Prokaryotic Communities on and Below the Seafloor 24 in the Back-Arc Hydrothermal System of the Southern Mariana Trough
View metadata, citation and similar papers at core.ac.uk brought to you by CORE provided by Springer - Publisher Connector Intra-Field Variation of Prokaryotic Communities On and Below the Seafloor 24 in the Back-Arc Hydrothermal System of the Southern Mariana Trough Shingo Kato, Moriya Ohkuma, and Akihiko Yamagishi Abstract Deep-sea hydrothermal vents harbor diverse prokaryotes. There are a variety of habitat types in a deep-sea hydrothermal field, e.g., active and inactive chimneys, iron-rich mats, venting fluid and hydrothermal plume. Numerous studies have shown the diversity and composition of prokaryotic communities in individual habitats. However, it is still unclear whether and how the characteristics of prokaryotic communities in their respective habitats are different. Previously, we reported 16S rRNA genes in a variety of habitats, i.e., hydrothermally active and inactive chimneys, iron-rich mats, a vent fluid, crustal fluids from boreholes, as well as ambient seawater in a back-arc basin hydrothermal field of the Southern Mariana Trough. Here we summarize the prokaryotic communities in the col- lected samples at higher taxonomic resolution (up to family level) using the detected 16S rRNA gene sequences and compare them using recently developed bioinformatics tools. The comparative analysis clearly highlights differences in prokaryotic communities among the habitat types on and below the seafloor in the Southern Mariana Trough. Furthermore, descriptions of cultured species and environmental clones close to the detected sequences provide valuable information for understanding of their distribution and potential of ecological roles in deep-sea hydrothermal fields. Keywords 16S rRNA gene Archaea Back-arc basin Bacteria Chemosynthetic ecosystem Deep- sea hydrothermal vents Prokaryotic community 24.1 Introduction The online version of this chapter (doi:10.1007/978-4-431-54865-2_24) Deep-sea hydrothermal vents are oases for organisms on the contains supplementary material, which is available to authorized users. -
Marine Extremophiles: a Source of Hydrolases for Biotechnological Applications
Mar. Drugs 2015, 13, 1925-1965; doi:10.3390/md13041925 OPEN ACCESS marine drugs ISSN 1660-3397 www.mdpi.com/journal/marinedrugs Article Marine Extremophiles: A Source of Hydrolases for Biotechnological Applications Gabriel Zamith Leal Dalmaso 1,2, Davis Ferreira 3 and Alane Beatriz Vermelho 1,* 1 BIOINOVAR—Biotechnology laboratories: Biocatalysis, Bioproducts and Bioenergy, Institute of Microbiology Paulo de Góes, Federal University of Rio de Janeiro, Av. Carlos Chagas Filho, 373, 21941-902 Rio de Janeiro, Brazil; E-Mail: [email protected] 2 Graduate Program in Plant Biotechnology, Health and Science Centre, Federal University of Rio de Janeiro, Av. Carlos Chagas Filho, 373, 21941-902 Rio de Janeiro, Brazil 3 BIOINOVAR—Biotechnology Laboratories: Virus-Cell Interaction, Institute of Microbiology Paulo de Góes, Federal University of Rio de Janeiro, Av. Carlos Chagas Filho, 373, 21941-902 Rio de Janeiro, Brazil; E-Mail: [email protected] * Author to whom correspondence should be addressed; E-Mail: [email protected]; Tel.: +55-(21)-3936-6743; Fax: +55-(21)-2560-8344. Academic Editor: Kirk Gustafson Received: 1 December 2014 / Accepted: 25 March 2015 / Published: 3 April 2015 Abstract: The marine environment covers almost three quarters of the planet and is where evolution took its first steps. Extremophile microorganisms are found in several extreme marine environments, such as hydrothermal vents, hot springs, salty lakes and deep-sea floors. The ability of these microorganisms to support extremes of temperature, salinity and pressure demonstrates their great potential for biotechnological processes. Hydrolases including amylases, cellulases, peptidases and lipases from hyperthermophiles, psychrophiles, halophiles and piezophiles have been investigated for these reasons. -
Aciduliprofundum Boonei’’, a Cultivated Obligate Thermoacidophilic Euryarchaeote from Deep-Sea Hydrothermal Vents
Extremophiles (2008) 12:119–124 DOI 10.1007/s00792-007-0111-0 ORIGINAL PAPER Tetraether membrane lipids of Candidatus ‘‘Aciduliprofundum boonei’’, a cultivated obligate thermoacidophilic euryarchaeote from deep-sea hydrothermal vents Stefan Schouten Æ Marianne Baas Æ Ellen C. Hopmans Æ Anna-Louise Reysenbach Æ Jaap S. Sinninghe Damste´ Received: 11 June 2007 / Accepted: 28 August 2007 / Published online: 28 September 2007 Ó Springer 2007 Abstract The lipid composition of Candidatus ‘‘Acidu- vents contain ecosystems, which are predominantly fueled liprofundum boonei’’, the only cultivated representative of by geochemical energy and are host to many newly archaea falling in the DHVE2 phylogenetic cluster, a group described free-living microbes, which are often associated of microorganisms ubiquitously occurring at hydrothermal with actively venting porous deep-sea vent deposits or vents, was studied. The predominant core membrane lipids ‘‘chimneys’’. The steep chemical and thermal gradients in this thermophilic euryarchaeote were found to be com- within the walls of these deposits provide a wide range of posed of glycerol dibiphytanyl glycerol tetraethers microhabitats for microorganisms with suitable conditions (GDGTs) containing 0–4 cyclopentyl moieties. In addition, for aerobic and anaerobic thermophiles and mesophiles GDGTs with an additional covalent bond between the (e.g. McCollom and Schock 1997). Indeed, both culture- isoprenoid hydrocarbon chains, so-called H-shaped dependent and -independent approaches have exposed a GDGTs, were present. The latter core lipids have been vast diversity of Bacteria and Archaea associated with rarely reported previously. Intact polar lipid analysis deep-sea vent deposits (e.g. Reysenbach and Shock 2002; revealed that they predominantly consist of GDGTs with a Schrenk et al. -
Phylogeny and Taxonomy of Archaea: a Comparison of the Whole-Genome-Based Cvtree Approach with 16S Rrna Sequence Analysis
Life 2015, 5, 949-968; doi:10.3390/life5010949 OPEN ACCESS life ISSN 2075-1729 www.mdpi.com/journal/life Article Phylogeny and Taxonomy of Archaea: A Comparison of the Whole-Genome-Based CVTree Approach with 16S rRNA Sequence Analysis Guanghong Zuo 1, Zhao Xu 2 and Bailin Hao 1;* 1 T-Life Research Center and Department of Physics, Fudan University, 220 Handan Road, Shanghai 200433, China; E-Mail: [email protected] 2 Thermo Fisher Scientific, 200 Oyster Point Blvd, South San Francisco, CA 94080, USA; E-Mail: [email protected] * Author to whom correspondence should be addressed; E-Mail: [email protected]; Tel.: +86-21-6565-2305. Academic Editors: Roger A. Garrett, Hans-Peter Klenk and Michael W. W. Adams Received: 9 December 2014 / Accepted: 9 March 2015 / Published: 17 March 2015 Abstract: A tripartite comparison of Archaea phylogeny and taxonomy at and above the rank order is reported: (1) the whole-genome-based and alignment-free CVTree using 179 genomes; (2) the 16S rRNA analysis exemplified by the All-Species Living Tree with 366 archaeal sequences; and (3) the Second Edition of Bergey’s Manual of Systematic Bacteriology complemented by some current literature. A high degree of agreement is reached at these ranks. From the newly proposed archaeal phyla, Korarchaeota, Thaumarchaeota, Nanoarchaeota and Aigarchaeota, to the recent suggestion to divide the class Halobacteria into three orders, all gain substantial support from CVTree. In addition, the CVTree helped to determine the taxonomic position of some newly sequenced genomes without proper lineage information. A few discrepancies between the CVTree and the 16S rRNA approaches call for further investigation. -
Marine Extremophiles: a Source of Hydrolases for Biotechnological Applications
Mar. Drugs 2015, 13, 1925-1965; doi:10.3390/md13041925 OPEN ACCESS marine drugs ISSN 1660-3397 www.mdpi.com/journal/marinedrugs Review Marine Extremophiles: A Source of Hydrolases for Biotechnological Applications Gabriel Zamith Leal Dalmaso 1,2, Davis Ferreira 3 and Alane Beatriz Vermelho 1,* 1 BIOINOVAR—Biotechnology laboratories: Biocatalysis, Bioproducts and Bioenergy, Institute of Microbiology Paulo de Góes, Federal University of Rio de Janeiro, Av. Carlos Chagas Filho, 373, 21941-902 Rio de Janeiro, Brazil; E-Mail: [email protected] 2 Graduate Program in Plant Biotechnology, Health and Science Centre, Federal University of Rio de Janeiro, Av. Carlos Chagas Filho, 373, 21941-902 Rio de Janeiro, Brazil 3 BIOINOVAR—Biotechnology Laboratories: Virus-Cell Interaction, Institute of Microbiology Paulo de Góes, Federal University of Rio de Janeiro, Av. Carlos Chagas Filho, 373, 21941-902 Rio de Janeiro, Brazil; E-Mail: [email protected] * Author to whom correspondence should be addressed; E-Mail: [email protected]; Tel.: +55-(21)-3936-6743; Fax: +55-(21)-2560-8344. Academic Editor: Kirk Gustafson Received: 1 December 2014 / Accepted: 25 March 2015 / Published: 3 April 2015 Abstract: The marine environment covers almost three quarters of the planet and is where evolution took its first steps. Extremophile microorganisms are found in several extreme marine environments, such as hydrothermal vents, hot springs, salty lakes and deep-sea floors. The ability of these microorganisms to support extremes of temperature, salinity and pressure demonstrates their great potential for biotechnological processes. Hydrolases including amylases, cellulases, peptidases and lipases from hyperthermophiles, psychrophiles, halophiles and piezophiles have been investigated for these reasons. -
Lists of Names of Prokaryotic Candidatus Taxa
NOTIFICATION LIST: CANDIDATUS LIST NO. 1 Oren et al., Int. J. Syst. Evol. Microbiol. DOI 10.1099/ijsem.0.003789 Lists of names of prokaryotic Candidatus taxa Aharon Oren1,*, George M. Garrity2,3, Charles T. Parker3, Maria Chuvochina4 and Martha E. Trujillo5 Abstract We here present annotated lists of names of Candidatus taxa of prokaryotes with ranks between subspecies and class, pro- posed between the mid- 1990s, when the provisional status of Candidatus taxa was first established, and the end of 2018. Where necessary, corrected names are proposed that comply with the current provisions of the International Code of Nomenclature of Prokaryotes and its Orthography appendix. These lists, as well as updated lists of newly published names of Candidatus taxa with additions and corrections to the current lists to be published periodically in the International Journal of Systematic and Evo- lutionary Microbiology, may serve as the basis for the valid publication of the Candidatus names if and when the current propos- als to expand the type material for naming of prokaryotes to also include gene sequences of yet-uncultivated taxa is accepted by the International Committee on Systematics of Prokaryotes. Introduction of the category called Candidatus was first pro- morphology, basis of assignment as Candidatus, habitat, posed by Murray and Schleifer in 1994 [1]. The provisional metabolism and more. However, no such lists have yet been status Candidatus was intended for putative taxa of any rank published in the journal. that could not be described in sufficient details to warrant Currently, the nomenclature of Candidatus taxa is not covered establishment of a novel taxon, usually because of the absence by the rules of the Prokaryotic Code. -
Post-Genomic Characterization of Metabolic Pathways in Sulfolobus Solfataricus
Post-Genomic Characterization of Metabolic Pathways in Sulfolobus solfataricus Jasper Walther Thesis committee Thesis supervisors Prof. dr. J. van der Oost Personal chair at the laboratory of Microbiology Wageningen University Prof. dr. W. M. de Vos Professor of Microbiology Wageningen University Other members Prof. dr. W.J.H. van Berkel Wageningen University Prof. dr. V.A.F. Martins dos Santos Wageningen University Dr. T.J.G. Ettema Uppsala University, Sweden Dr. S.V. Albers Max Planck Institute for Terrestrial Microbiology, Marburg, Germany This research was conducted under the auspices of the Graduate School VLAG Post-Genomic Characterization of Metabolic Pathways in Sulfolobus solfataricus Jasper Walther Thesis Submitted in fulfilment of the requirements for the degree of doctor at Wageningen University by the authority of the Rector Magnificus Prof. dr. M.J. Kropff, in the presence of the Thesis Committee appointed by the Academic Board to be defended in public on Monday 23 January 2012 at 11 a.m. in the Aula. Jasper Walther Post-Genomic Characterization of Metabolic Pathways in Sulfolobus solfataricus, 164 pages. Thesis, Wageningen University, Wageningen, NL (2012) With references, with summaries in Dutch and English ISBN 978-94-6173-203-3 Table of contents Chapter 1 Introduction 1 Chapter 2 Hot Transcriptomics 17 Chapter 3 Reconstruction of central carbon metabolism in Sulfolobus solfataricus using a two-dimensional gel electrophoresis map, stable isotope labelling and DNA microarray analysis 45 Chapter 4 Identification of the Missing -
Calditol-Linked Membrane Lipids Are Required for Acid Tolerance in Sulfolobus Acidocaldarius
Calditol-linked membrane lipids are required for acid tolerance in Sulfolobus acidocaldarius Zhirui Zenga,1, Xiao-Lei Liub,1, Jeremy H. Weia, Roger E. Summonsc, and Paula V. Welandera,2 aDepartment of Earth System Science, Stanford University, Stanford, CA 94305; bDepartment of Geology and Geophysics, University of Oklahoma, Norman, OK 73019; and cDepartment of Earth, Atmospheric and Planetary Sciences, Massachusetts Institute of Technology, Cambridge, MA 02139 Edited by Katherine H. Freeman, Pennsylvania State University, University Park, PA, and approved November 7, 2018 (received for review August 14, 2018) Archaea have many unique physiological features of which the lipid and sea-surface temperatures in the Mesozoic and early Cenozoic, composition of their cellular membranes is the most striking. and the geologic history of archaea in ancient sediments (13, 14). Archaeal ether-linked isoprenoidal membranes can occur as bilayers However, deciphering the evolutionary implications of archaeal or monolayers, possess diverse polar head groups, and a multiplicity lipid biosynthesis, as well as being able to properly interpret ar- of ring structures in the isoprenoidal cores. These lipid structures chaeal lipid biomarkers, requires a full understanding of the are proposed to provide protection from the extreme temperature, biosynthetic pathways and the physiological roles of these various pH, salinity, and nutrient-starved conditions that many archaea structures in extant archaea. inhabit. However, many questions remain regarding the synthesis Studies of archaeal membrane lipid synthesis have revealed and physiological role of some of the more complex archaeal lipids. distinct proteins and biochemical reactions, but several open In this study, we identify a radical S-adenosylmethionine (SAM) questions remain (4, 15). -
Diversity of Archaea Domain in Cuatro Cienegas Basin: Archaean Domes
bioRxiv preprint doi: https://doi.org/10.1101/766709; this version posted September 12, 2019. The copyright holder for this preprint (which was not certified by peer review) is the author/funder, who has granted bioRxiv a license to display the preprint in perpetuity. It is made available under aCC-BY-NC-ND 4.0 International license. 1 Diversity of Archaea Domain in Cuatro Cienegas Basin: Archaean Domes 2 3 Medina-Chávez Nahui Olin1, Viladomat-Jasso Mariette2, Olmedo-Álvarez Gabriela3, Eguiarte Luis 4 E2, Souza Valeria2, De la Torre-Zavala Susana1,4 5 6 1Universidad Autónoma de Nuevo León, Facultad de Ciencias Biológicas, Instituto de 7 Biotecnología. Av. Pedro de Alba S/N Ciudad Universitaria. San Nicolás de los Garza, Nuevo León, 8 México. C.P. 66455. 9 2Instituto de Ecología, UNAM, Circuito Exterior S/N anexo Jardín Botánico exterior. Ciudad 10 Universitaria, Ciudad de México, C.P. 04500 11 3Departamento de Ingeniería Genética, Centro de Investigación y de Estudios Avanzados del I.P.N. 12 Campus Guanajuato, AP 629 Irapuato, Guanajuato 36500, México 13 14 4Correspondence should be addressed to Susana De la Torre-Zavala; 15 [email protected]. 16 17 18 19 20 21 22 1 bioRxiv preprint doi: https://doi.org/10.1101/766709; this version posted September 12, 2019. The copyright holder for this preprint (which was not certified by peer review) is the author/funder, who has granted bioRxiv a license to display the preprint in perpetuity. It is made available under aCC-BY-NC-ND 4.0 International license. 23 Abstract 24 Herein we describe the Archaea diversity in a shallow pond in the Cuatro Ciénegas Basin (CCB), 25 Northeast Mexico, with fluctuating hypersaline conditions containing elastic microbial mats that 26 can form small domes where their anoxic inside reminds us of the characteristics of the Archaean 27 Eon, rich in methane and sulfur gases; thus, we named this site the Archaean Domes (AD). -
4 Metabolic and Taxonomic Diversification in Continental Magmatic Hydrothermal Systems
Maximiliano J. Amenabar, Matthew R. Urschel, and Eric S. Boyd 4 Metabolic and taxonomic diversification in continental magmatic hydrothermal systems 4.1 Introduction Hydrothermal systems integrate geological processes from the deep crust to the Earth’s surface yielding an extensive array of spring types with an extraordinary diversity of geochemical compositions. Such geochemical diversity selects for unique metabolic properties expressed through novel enzymes and functional characteristics that are tailored to the specific conditions of their local environment. This dynamic interaction between geochemical variation and biology has played out over evolu- tionary time to engender tightly coupled and efficient biogeochemical cycles. The timescales by which these evolutionary events took place, however, are typically in- accessible for direct observation. This inaccessibility impedes experimentation aimed at understanding the causative principles of linked biological and geological change unless alternative approaches are used. A successful approach that is commonly used in geological studies involves comparative analysis of spatial variations to test ideas about temporal changes that occur over inaccessible (i.e. geological) timescales. The same approach can be used to examine the links between biology and environment with the aim of reconstructing the sequence of evolutionary events that resulted in the diversity of organisms that inhabit modern day hydrothermal environments and the mechanisms by which this sequence of events occurred. By combining molecu- lar biological and geochemical analyses with robust phylogenetic frameworks using approaches commonly referred to as phylogenetic ecology [1, 2], it is now possible to take advantage of variation within the present – the distribution of biodiversity and metabolic strategies across geochemical gradients – to recognize the extent of diversity and the reasons that it exists. -
Microbes from Hell
Microbes from Hell Microbes from Hell Patrick Forterre Translated by Teresa Lavender Fagan The University of Chicago Press Chicago and London The University of Chicago Press, Chicago 60637 The University of Chicago Press, Ltd., London © 2016 by The University of Chicago All rights reserved. Published 2016. Printed in the United States of America Originally published as Microbes de l’enfer by Patrick Forterre. © Editions Belin, 2007. 25 24 23 22 21 20 19 18 17 16 1 2 3 4 5 ISBN- 13: 978- 0- 226- 26582- 7 (cloth) ISBN- 13: 978- 0- 226- 26596- 4 (e- book) DOI: 10.7208/chicago/9780226265964.001.0001 Library of Congress Cataloging- in- Publication Data Names: Forterre, Patrick, author. | Fagan, Teresa Lavender, translator. Title: Microbes from hell / Patrick Forterre ; translated by Teresa Lavender Fagan. Other titles: Microbes de l’enfer. English Description: Chicago ; London : University of Chicago Press, 2016. | © 2016 | Includes bibliographical references and index. Identifiers: LCCN 2016009697 | ISBN 9780226265827 (cloth : alkaline paper) | ISBN 9780226265964 (e- book) Subjects: LCSH: Thermophilic microorganisms. | Microorganisms— Effect of heat on. Classification: LCC QR84.8.F6713 2016 | DDC 579.3/17— dc23 LC record available at http:// lccn .loc .gov /2016009697 ♾ This paper meets the requirements of ANSI/NISO Z39.48- 1992 (Permanence of Paper). Children of steam and scalded rock, a story you have to tell, Writ in the glare of sunshine bright, Sculptured and etched in marble white, Illuminated in colors bold, Richer than ever parchment old, Children of steam and scalded rock, what is the story you have to tell? Our legends are old, of greater age than the mountains round about. -
An Integrated Study Reveals Diverse Methanogens, Thaumarchaeota, and Yet-Uncultivated Archaeal Lineages in Armenian Hot Springs
Antonie van Leeuwenhoek DOI 10.1007/s10482-013-9927-z ORIGINAL PAPER An integrated study reveals diverse methanogens, Thaumarchaeota, and yet-uncultivated archaeal lineages in Armenian hot springs Brian P. Hedlund • Jeremy A. Dodsworth • Jessica K. Cole • Hovik H. Panosyan Received: 27 February 2013 / Accepted: 20 April 2013 Ó Springer Science+Business Media Dordrecht 2013 Abstract Culture-independent and enrichment tech- spring in Arzakan was colonized by a photosynthetic niques, with an emphasis on members of the Archaea, mat dominated by Cyanobacteria, in addition to were used to determine the composition and structure Proteobacteria, Bacteroidetes, Chloroflexi, Spiro- of microbial communities inhabiting microbial mats in chaeta and a diversity of other Bacteria. The spring the source pools of two geothermal springs near the in Jermuk was colonized by phylotypes related to towns of Arzakan and Jermuk in Armenia. Amplifi- sulfur, iron, and hydrogen chemolithotrophs in the cation of small-subunit rRNA genes using ‘‘universal’’ Betaproteobacteria and Epsilonproteobacteria, along primers followed by pyrosequencing (pyrotags) with a diversity of other Bacteria. Analysis of near revealed highly diverse microbial communities in full-length small subunit rRNA genes amplified using both springs, with [99 % of pyrosequences corre- Archaea-specific primers showed that both springs are sponding to members of the domain Bacteria. The inhabited by a diversity of methanogens, including Methanomicrobiales and Methanosarcinales and rel- atives of Methanomassiliicoccus luminyensis, close relatives of the ammonia-oxidizing archaeon (AOA) Electronic supplementary material The online version of ‘‘Candidatus Nitrososphaera gargensis’’, and the yet- this article (doi:10.1007/s10482-013-9927-z) contains supplementary material, which is available to authorized users.