Episodic Tremor and Slip Explained by Fluid-Enhanced Microfracturing and Sealing Maxime Bernaudin, Frederic Gueydan
Total Page:16
File Type:pdf, Size:1020Kb
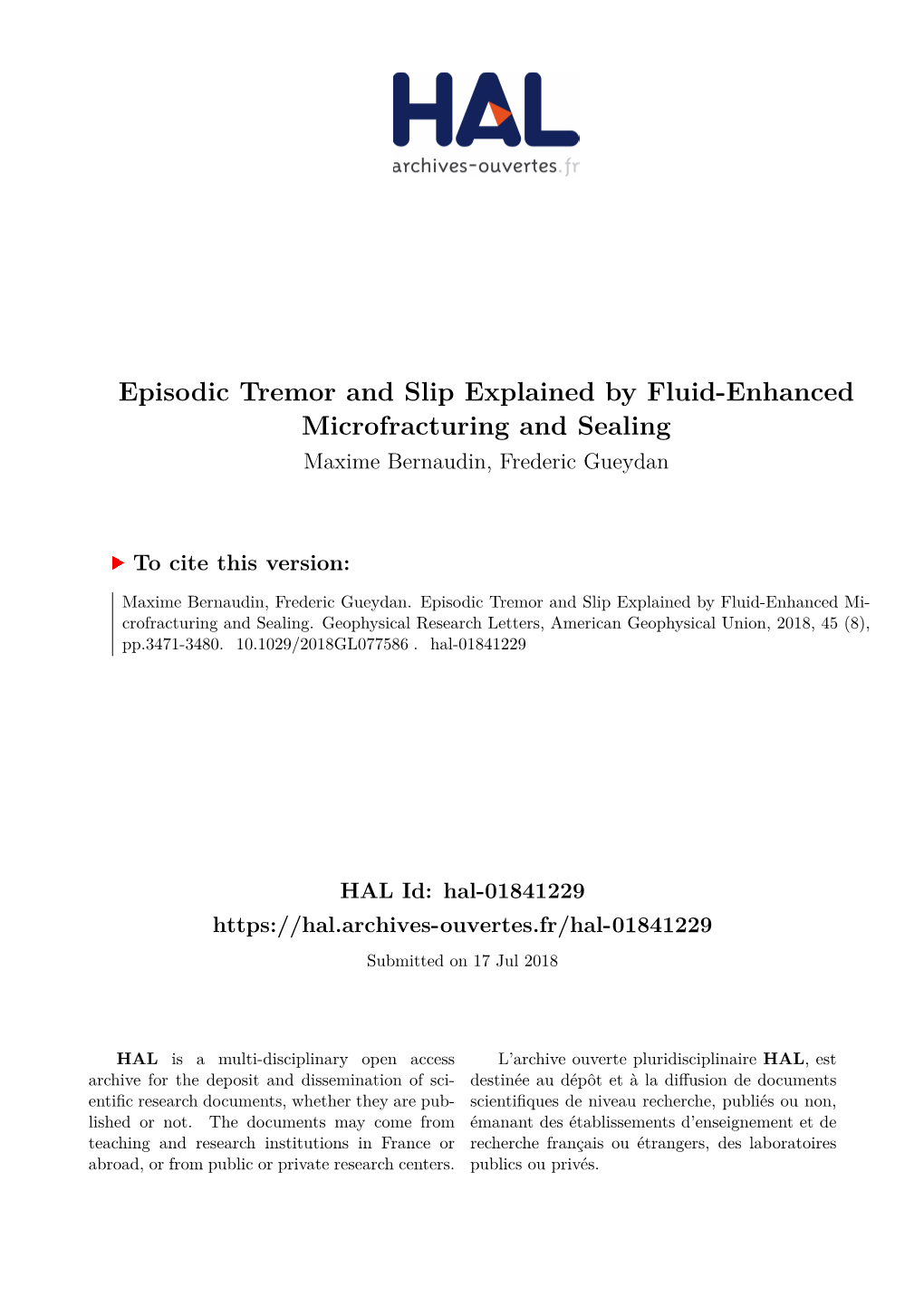
Load more
Recommended publications
-
Seismic Wave Triggering of Nonvolcanic Tremor, Episodic Tremor and Slip, and Earthquakes on Vancouver Island Justin L
JOURNAL OF GEOPHYSICAL RESEARCH, VOL. 114, B00A01, doi:10.1029/2008JB005875, 2009 Click Here for Full Article Seismic wave triggering of nonvolcanic tremor, episodic tremor and slip, and earthquakes on Vancouver Island Justin L. Rubinstein,1,2 Joan Gomberg,3 John E. Vidale,1 Aaron G. Wech,1 Honn Kao,4 Kenneth C. Creager,1 and Garry Rogers4 Received 16 June 2008; revised 14 October 2008; accepted 20 November 2008; published 19 February 2009. [1] We explore the physical conditions that enable triggering of nonvolcanic tremor and earthquakes by considering local seismic activity on Vancouver Island, British Columbia during and immediately after the arrival of large-amplitude seismic waves from 30 teleseismic and 17 regional or local earthquakes. We identify tremor triggered by four of the teleseismic earthquakes. The close temporal and spatial proximity of triggered tremor to ambient tremor and aseismic slip indicates that when a fault is close to or undergoing failure, it is particularly susceptible to triggering of further events. The amplitude of the triggering waves also influences the likelihood of triggering both tremor and earthquakes such that large amplitude waves triggered tremor in the absence of detectable aseismic slip or ambient tremor. Tremor and energy radiated from regional/local earthquakes share the same frequency passband so that tremor cannot be identified during these smaller, more frequent events. We confidently identify triggered local earthquakes following only one teleseism, that with the largest amplitude, and four regional or local events that generated vigorous aftershock sequences in their immediate vicinity. Earthquakes tend to be triggered in regions different from tremor and with high ambient seismicity rates. -
Sparsity-Based Estimates of Slow Slip Distributions in Cascadia
United States Geological Survey Earthquake Hazards Program Final Technical Report USGS Award Number G17AP00026 Sparsity-based estimates of slow slip distributions in Cascadia Author: John Loveless Department of Geosciences Smith College Clark Science Center 44 College Lane Northampton, MA 01063, USA Phone: (413) 585-2657 Fax: (413) 585-3786 Email: [email protected] Other personnel: Elias Molitors Bergman, Smith College Post-baccalaureate Research Assistant Sofia Johnson, Smith College Summer Undergraduate Research Fellow Eileen Evans, United States Geological Survey Collaborator Award Term: January 1, 2017–December 31, 2017 ABSTRACT Since the first geodetic detection of slow slip events (SSEs) on the Cascadia Subduction Zone in the 1990s, the role that these events play in the seismic moment balance of the plate boundary zone has been intensely studied. Slip during the events has been estimated to take place below the portion of the subduction zone that is inferred to be interseismically coupled and therefore most likely to generate future large earthquakes. However, the degree of spatial separation between the coupled and/or seismogenic zone, and the slowly slipping zone is ambiguous, in part because of the smoothing-based regularization technique that has been employed in many inversions of geodetic displacement fields for slow slip distributions. In this funded research, we compare two regularization strategies in estimating the distribution of slow slip: the classic smoothing-based approach and total variation regularization (TVR), which seeks spatial clusters of homogeneous slip, the magnitude of which may vary abruptly from patch to patch. Whereas smoothing inherently blurs the spatial distribution of estimated slow slip, and hence the degree to which that distribution may overlap with patterns of coupling and/or coseismic slip, TVR enables imaging of spatially distinct regions of slip behaviors. -
Relation of Slow Slip Events to Subsequent Earthquake Rupture
Earthquake and tsunami forecasts: Relation of slow slip events to subsequent earthquake rupture Timothy H. Dixona,1, Yan Jiangb, Rocco Malservisia, Robert McCaffreyc, Nicholas Vossa, Marino Prottid, and Victor Gonzalezd aSchool of Geosciences, University of South Florida, Tampa, FL 33620; bPacific Geoscience Centre, Geological Survey of Canada, BC, Canada V8L 4B2; cDepartment of Geology, Portland State University, Portland, OR 97201; and dObservatorio Vulcanológico y Sismológico de Costa Rica, Universidad Nacional, Heredia 3000, Costa Rica Edited* by David T. Sandwell, Scripps Institution of Oceanography, La Jolla, CA, and approved October 24, 2014 (received for review June 30, 2014) The 5 September 2012 Mw 7.6 earthquake on the Costa Rica sub- Geologic and Seismic Background duction plate boundary followed a 62-y interseismic period. High- The Nicoya Peninsula forms the western edge of the Caribbean precision GPS recorded numerous slow slip events (SSEs) in the plate, where the Cocos plate subducts beneath the Caribbean decade leading up to the earthquake, both up-dip and down-dip plate along the Middle American Trench at about 8 cm/y (3). The of seismic rupture. Deeper SSEs were larger than shallower ones region has a well-defined earthquake cycle, with large (M > 7) and, if characteristic of the interseismic period, release most lock- earthquakes in 1853, 1900, 1950 (M 7.7), and most recently 5 ing down-dip of the earthquake, limiting down-dip rupture and September 2012 (Mw 7.6). Smaller (M ∼ 7) events in 1978 and earthquake magnitude. Shallower SSEs were smaller, accounting 1990 have also occurred nearby (4). Large tsunamis have not for some but not all interseismic locking. -
Episodic Tremor and Slip in the Pacific Northwest
winter/spring 2007 featured science: Episodic Tremor and Slip in the Pacific Northwest Every 14 months the Pacific Northwest experiences slow slip on a aultf that is the equivalent of about a magnitude 6.5 earthquake. While a typical earthquake of this magnitude happens in less than 10 seconds, the duration of these slip events is two to several weeks. The most recent event occurred from January 14 through February 1, 2007. In the Pacific Northwest, the Juan de onSitenewsletter Fuca plate is subducting (or dipping) beneath the North American plate from northern California From The Principal Investigators to Vancouver Island. EarthScope’s multi-disciplinary approach These plates slide past to improving our understanding of the structure each other along the and formation of continents and the physical solid green, dashed Figure 1. Courtesy of H. Dragert, Geological Survey of Canada processes that control earthquakes and yellow and dashed red lines in Figure 1. The Cascadia subduction zone, as it is volcanoes has spurred the development of new called, experiences large earthquakes, perhaps as large as the 2004 Sumatra techniques for analyzing and interpreting many earthquake, once every 500 years on average. The last one was about 300 years types of geophysical data. Throughout this issue, ago in January 1700. The slip during these earthquakes, occurring on the “locked” we highlight some of EarthScope’s integrated zone in the figure, is thought to accommodate most, if not all, of the relative activities. motion between the North American plate and the Juan de Fuca plate. Down dip of this locked zone (red dashed lines), the plates must still slide past each In our science feature, two EarthScope researchers, Ken Creager of the University of other. -
Cascadia Low Frequency Earthquakes at the Base of an Overpressured Subduction Shear Zone ✉ Andrew J
ARTICLE https://doi.org/10.1038/s41467-020-17609-3 OPEN Cascadia low frequency earthquakes at the base of an overpressured subduction shear zone ✉ Andrew J. Calvert 1 , Michael G. Bostock 2, Geneviève Savard 3 & Martyn J. Unsworth4 In subduction zones, landward dipping regions of low shear wave velocity and elevated Poisson’s ratio, which can extend to at least 120 km depth, are interpreted to be all or part of the subducting igneous oceanic crust. This crust is considered to be overpressured, because fl 1234567890():,; uids within it are trapped beneath an impermeable seal along the overlying inter-plate boundary. Here we show that during slow slip on the plate boundary beneath southern Vancouver Island, low frequency earthquakes occur immediately below both the landward dipping region of high Poisson’s ratio and a 6–10 km thick shear zone revealed by seismic reflections. The plate boundary here either corresponds to the low frequency earthquakes or to the anomalous elastic properties in the lower 3–5 km of the shear zone immediately above them. This zone of high Poisson’s ratio, which approximately coincides with an electrically conductive layer, can be explained by slab-derived fluids trapped at near-lithostatic pore pressures. 1 Department of Earth Sciences, Simon Fraser University, 8888 University Drive, Burnaby, BC V5A 1S6, Canada. 2 Department of Earth, Ocean and Atmospheric Sciences, 2207 Main Mall, University of British Columbia, Vancouver, BC V6T 1Z4, Canada. 3 Department of Geosciences, University of Calgary, 2500 University Drive NW, Calgary, AB T2N 1N4, Canada. 4 Department of Physics, University of Alberta, Edmonton, AB T6G 2E9, Canada. -
Model for Triggering of Non-Volcanic Tremor by Earthquakes
Wright State University CORE Scholar Physics Faculty Publications Physics 2012 Model for Triggering of Non-Volcanic Tremor by Earthquakes Naum I. Gershenzon Wright State University - Main Campus, [email protected] Gust Bambakidis Wright State University - Main Campus, [email protected] Follow this and additional works at: https://corescholar.libraries.wright.edu/physics Part of the Physics Commons Repository Citation Gershenzon, N. I., & Bambakidis, G. (2012). Model for Triggering of Non-Volcanic Tremor by Earthquakes. https://corescholar.libraries.wright.edu/physics/552 This Article is brought to you for free and open access by the Physics at CORE Scholar. It has been accepted for inclusion in Physics Faculty Publications by an authorized administrator of CORE Scholar. For more information, please contact [email protected]. Model for triggering of non-volcanic tremor by earthquakes Naum I. Gershenzon1,2, Gust Bambakidis1 1Physics Department, Wright State University, 3640 Colonel Glenn Highway Dayton, OH 45435 2Department of Earth and Environmental Sciences, Wright State University, 3640 Colonel Glenn Highway Dayton, OH 45435 [1] There is evidence of tremor triggering by seismic waves emanating from distant large earthquakes. The frequency content of both triggered and ambient tremor are largely identical, suggesting that this property does not depend directly on the nature of the source. We show here that the model of plate dynamics developed earlier by us is an appropriate tool for describing tremor triggering. In the framework of this model, tremor is an internal response of a fault to a failure triggered by external disturbances. The model predicts generation of radiation in a frequency range defined by the fault parameters. -
Geodetic and Seismic Signatures of Episodic Tremor and Slip in the Northern Cascadia Subduction Zone
Earth Planets Space, 56, 1143–1150, 2004 Geodetic and seismic signatures of episodic tremor and slip in the northern Cascadia subduction zone H. Dragert, K. Wang, and G. Rogers Geological Survey of Canada, Pacific Geoscience Centre, Sidney, B.C., V8L 4B2, Canada (Received June 2, 2004; Revised December 2, 2004; Accepted December 24, 2004) Slip events with an average duration of about 10 days and effective total slip displacements of severalc entimetres have been detected on the deeper (25 to 45 km) part of the northern Cascadia subduction zone interface by observing transient surface deformation on a network of continuously recording Global Positioning System (GPS) sites. The slip events occur down-dip from the currently locked, seismogenic portion of the subduction zone, and, for the geographic region around Victoria, British Columbia, repeat at 13 to 16 month intervals. These episodes of slip are accompanied by distinct, low-frequency tremors, similar to those reported in the forearc region of southern Japan. Although the processes which generate this phenomenon of episodic tremor and slip (ETS) are not well understood, it is possible that the ETS zone may constrain the landward extent of megathrust rupture, and conceivable that an ETS event could precede the next great thrust earthquake. Key words: Crustal deformation, GPS, slow earthquakes, non-volcanic tremors, Cascadia margin. 1. Introduction of continuous GPS sites in northern Cascadia are marked by Beginning in 1992, increasing numbers of automated numerous, brief, episodic reversals. This is best illustrated continuous Global Positioning System (GPS) sites have by the east-component time series at the Victoria GPS site been established in southwestern British Columbia and (ALBH) where the motion relative to DRAO is clearly char- northwestern United States in order to monitor crustal mo- acterized by a sloped saw-tooth function: For periods of 13 tions due to present-day tectonics. -
Slow Earthquakes: an Overview
SLOW EARTHQUAKES: AN OVERVIEW THEORETICAL SEISMOLOGY AIDA QUEZADA-REYES Slow Earthquakes: An Overview ABSTRACT Slow earthquakes have been observed in California and Japan. This type of earthquakes is characterized by nearly exponential strain changes that last for hours to days. Studies show that the duration of these earthquakes ranges from a few seconds to a few hundred seconds. In some cases, these events are accompanied by non-volcanic tremor that suggests the presence of forced fluid flow. Slow earthquakes occurrence suggests that faults can sustain ruptures over different time scales, that they have a slow rupture propagation, a low slip rate or both. However, the loading of the seismogenic zone by slow earthquakes has not been yet fully understood. In this work, I present an overview of the causes that originate slow earthquakes and I also provide the Cascadia example of events that have been studied in the past. 1. INTRODUCTION Earthquakes occur as a consequence of a gradual stress buildup in a region that eventually exceeds some threshold value or critical local strength, greater than that the rock can withstand, and it generates a rupture. The resulting motion is related to a drop in shear stress. The rupture propagation may be controlled by the failure criterion, the constitutive properties and the initial conditions on the fault, such as the frictional properties of the fault surface or the stress distribution around it (Mikumo et. al., 2003). This rupture has been observed to be on the order of 10-1 to 105 m on micro- to large earthquakes and on the order of 10-3 to 1 m on laboratory-created rupture (Ohnaka, 2003). -
Moment Release Rate of Cascadia Tremor Constrained by GPS Ana C
JOURNAL OF GEOPHYSICAL RESEARCH, VOL. 114, B00A05, doi:10.1029/2008JB005909, 2009 Click Here for Full Article Moment release rate of Cascadia tremor constrained by GPS Ana C. Aguiar,1 Timothy I. Melbourne,1 and Craig W. Scrivner1 Received 1 July 2008; revised 19 February 2009; accepted 6 March 2009; published 9 July 2009. [1] A comparison of GPS and seismic analyses of 23 distinct episodic tremor and slip events, located throughout the Cascadia subduction zone over an 11-year period, yields a highly linear relationship between moment release, as estimated from GPS, and total duration of nonvolcanic tremor, as summed from regional seismic arrays. The events last 1–5 weeks, typically produce 5 mm of static forearc deformation, and show cumulative totals of tremor that range from 40 to 280 h. Moment released by each event is estimated by inverting GPS-measured deformation, which is sensitive to all rates of tremor-synchronous faulting, including aseismic creep, for total slip along the North American-Juan de Fuca plate interface. Tremor, which is shown to be largely invariant in amplitude and frequency content both between events and with respect to its duration, is quantified using several different parameterizations that agree to within 10%. All known Cascadia events detected since 1997, which collectively span the Cascadia arc from northern California to Vancouver Island, Canada, release moment during tremor at a rate of 5.2 ± 0.4 Â 1016 N m per hour of recorded tremor. This relationship enables estimation of moment dissipation, via seismic monitoring of tremor, along the deeper Cascadia subduction zone that poses the greatest threat to its major metropolitan centers. -
Episodic Tremor & Slip
Activity— ETS (Episodic Tremor & Slip) Tectonic processes in a subduction zone Episodic Tremor and Slip (ETS) is a process by which a portion of the accumulated elastic energy is released along a subduction zone plate boundary at intermediate depth. During ETS events on the Cascadia subduction zone, the Juan de Fuca Plate slips a few centimeters farther beneath the North American Plate over an interval from a few days to two weeks. This “slow slip” is accompanied by release of seismic waves in a “tremor”-like fashion that is much longer in duration and lower in frequency than seismic waves released by standard earthquakes.* Here’s the problem – if the slow earthquakes are relieving stress in the ‘slip’ zone, what’s happening in the locked zone? What some geologists are thinking is that any stress that is relieved in the slip zone is transferred to the locked zone! The locked zone is getting stress added to it from both sides! Grid 1: Locked Plates (contact between subducting oceanic and overlying continental plates) Grid 2: Episodic slip with accompanying tremor (coastal GPS stations are being pushed NE) No GPS movement; this area is above the “unlocked” contact between the plates. Science Standards (NGSS; pg. 287) • From Molecules to Organisms—Structures and Processes: MS-LS1-8 • Motion and Stability: Forces and Interactions Animation depicted above is HS-PS2-1, MS-PS2-2 • Energy: MS-PS3-1, MS-PS3-2, HS-PS3-2, on the CEETEP DVD in the folder: MS-PS3-5 3. Cascadia Earthquakes & Tsunamis • Waves and Their Applications in Technologies > 2. -
Fault Zone Heterogeneities Explain Depth-Dependent Pattern And
ARTICLE https://doi.org/10.1038/s41467-021-22232-x OPEN Fault zone heterogeneities explain depth- dependent pattern and evolution of slow earthquakes in Cascadia ✉ Yingdi Luo 1,2 & Zhen Liu 2 Slow earthquakes including tremor and slow-slip events are recent additions to the con- ventional earthquake family and have a close link to megathrust earthquakes. Slow earth- 1234567890():,; quakes along the Cascadia subduction zone display a diverse behavior at different spatiotemporal scales and an intriguing increase of events frequency with depth. However, what causes such variability, especially the depth-dependent behavior is not well understood. Here we build on a heterogeneous asperities-in-matrix fault model that incorporates differ- ential pore pressure in a rate-and-state friction framework to investigate the underlying processes of the observed episodic tremor and slow-slip (ETS) variability. We find that the variations of effective normal stress (pore pressure) is one important factor in controlling ETS behavior. Our model reproduces the full complexity of ETS patterns and the depth-frequency scaling that agree quantitatively well with observations, suggesting that fault zone hetero- geneities can be one viable mechanism to explain a broad spectrum of transient fault behaviors. 1 JIFRESSE, University of California, Los Angeles, CA, USA. 2 Jet Propulsion Laboratory, California Institute of Technology, Pasadena, CA, USA. ✉ email: [email protected] NATURE COMMUNICATIONS | (2021) 12:1959 | https://doi.org/10.1038/s41467-021-22232-x | www.nature.com/naturecommunications 1 ARTICLE NATURE COMMUNICATIONS | https://doi.org/10.1038/s41467-021-22232-x ecent advances in seismology and geodesy have led to many as the first-order approximation to characterize the ETS types at Rnew findings of unconventional slow earthquakes such as depth. -
Cascading Rupture of Patches of High Seismic Energy Release Controls The
Nakamoto et al. Earth, Planets and Space (2021) 73:59 https://doi.org/10.1186/s40623-021-01384-6 FULL PAPER Open Access Cascading rupture of patches of high seismic energy release controls the growth process of episodic tremor and slip events Keita Nakamoto1, Yoshihiro Hiramatsu2* , Takahiko Uchide3 and Kazutoshi Imanishi3 Abstract Slip phenomena on plate interfaces refect the heterogeneous physical properties of the slip plane and, thus, exhibit a wide variety of slip velocities and rupture propagation behaviors. Recent fndings on slow earthquakes reveal simi- larities and diferences between slow and regular earthquakes. Episodic tremor and slip (ETS) events, a type of slow earthquake widely observed in subduction zones, likewise show diverse activity. We investigated the growth of 17 ETS events beneath the Kii Peninsula in the Nankai subduction zone, Japan. Analyses of waveform data recorded by a seismic array enabled us to locate tremor hypocenters and estimate the migration patterns and spatial distribu- tion of the energy release of tremor events. Here, we describe three major features in the growth of ETS events. First, independent of their start point and migration pattern, ETS events exhibit patches of high seismic energy release on the up-dip part of the ETS zone, suggesting that the location of these patches is controlled by inherent physical or frictional properties of the plate interface. Second, ETS events usually start outside the high-energy patches, and their fnal extent depends on whether the patches participate in the rupture. Third, we recognize no size dependence in the initiation phase of ETS events of diferent sizes with comparable start points.