1 Transcription Factor Network Analysis Identifies REST/NRSF As an Intrinsic 2 Regulator of CNS Regeneration
Total Page:16
File Type:pdf, Size:1020Kb
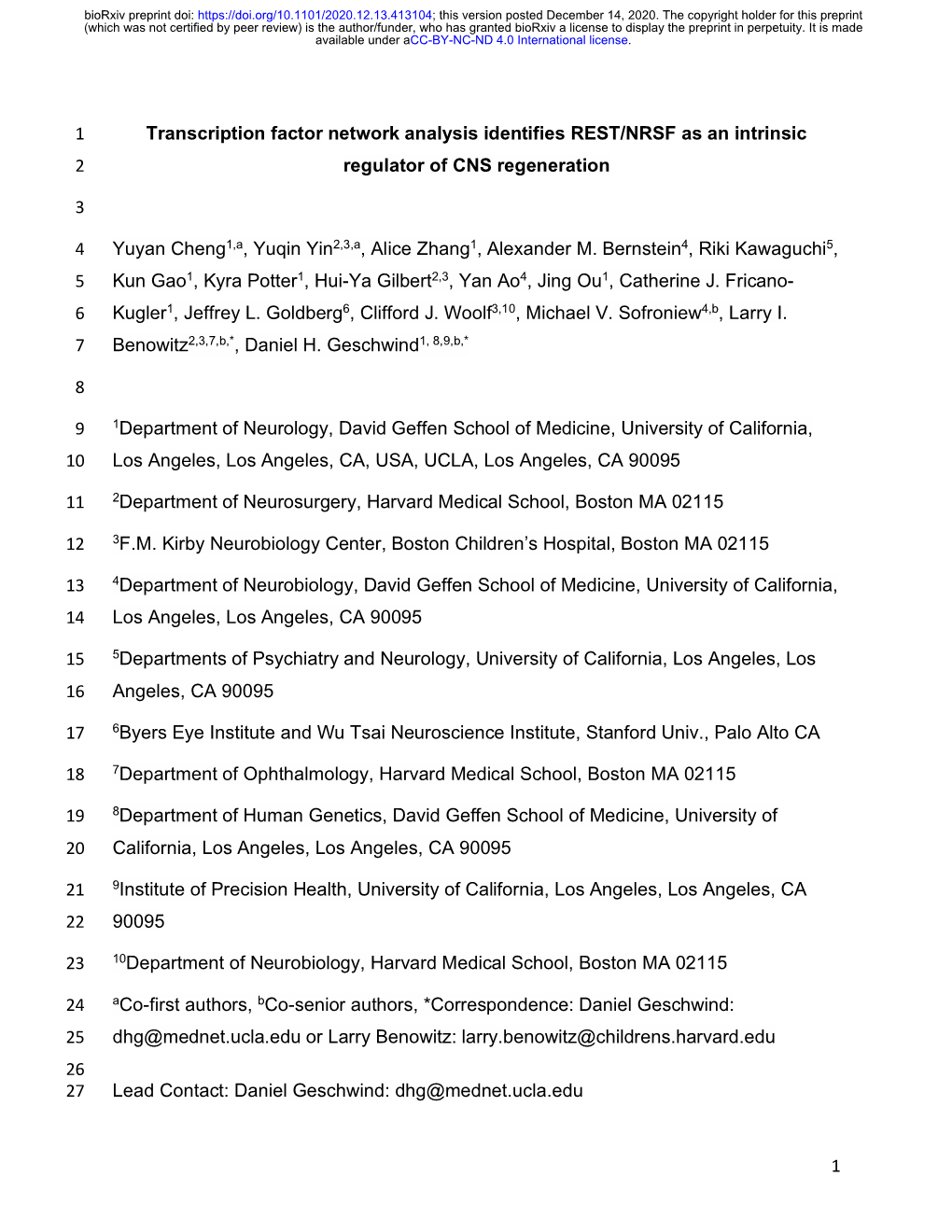
Load more
Recommended publications
-
RBP-J Signaling − Cells Through Notch Novel IRF8-Controlled
Sca-1+Lin−CD117− Mesenchymal Stem/Stromal Cells Induce the Generation of Novel IRF8-Controlled Regulatory Dendritic Cells through Notch −RBP-J Signaling This information is current as of September 25, 2021. Xingxia Liu, Shaoda Ren, Chaozhuo Ge, Kai Cheng, Martin Zenke, Armand Keating and Robert C. H. Zhao J Immunol 2015; 194:4298-4308; Prepublished online 30 March 2015; doi: 10.4049/jimmunol.1402641 Downloaded from http://www.jimmunol.org/content/194/9/4298 Supplementary http://www.jimmunol.org/content/suppl/2015/03/28/jimmunol.140264 http://www.jimmunol.org/ Material 1.DCSupplemental References This article cites 59 articles, 19 of which you can access for free at: http://www.jimmunol.org/content/194/9/4298.full#ref-list-1 Why The JI? Submit online. • Rapid Reviews! 30 days* from submission to initial decision by guest on September 25, 2021 • No Triage! Every submission reviewed by practicing scientists • Fast Publication! 4 weeks from acceptance to publication *average Subscription Information about subscribing to The Journal of Immunology is online at: http://jimmunol.org/subscription Permissions Submit copyright permission requests at: http://www.aai.org/About/Publications/JI/copyright.html Email Alerts Receive free email-alerts when new articles cite this article. Sign up at: http://jimmunol.org/alerts The Journal of Immunology is published twice each month by The American Association of Immunologists, Inc., 1451 Rockville Pike, Suite 650, Rockville, MD 20852 Copyright © 2015 by The American Association of Immunologists, Inc. All rights reserved. Print ISSN: 0022-1767 Online ISSN: 1550-6606. The Journal of Immunology Sca-1+Lin2CD1172 Mesenchymal Stem/Stromal Cells Induce the Generation of Novel IRF8-Controlled Regulatory Dendritic Cells through Notch–RBP-J Signaling Xingxia Liu,*,1 Shaoda Ren,*,1 Chaozhuo Ge,* Kai Cheng,* Martin Zenke,† Armand Keating,‡,x and Robert C. -
Neuroinflammation Triggered by Β-Glucan/Dectin-1 Signaling Enables CNS Axon Regeneration
Neuroinflammation triggered by β-glucan/dectin-1 signaling enables CNS axon regeneration Katherine T. Baldwina,b,1, Kevin S. Carbajalc,d,1, Benjamin M. Segalc,d,2, and Roman J. Gigera,b,c,d,2 aDepartment of Cell and Developmental Biology, bCellular and Molecular Biology Graduate Program, cNeuroscience Graduate Program, and dHoltom-Garrett Program in Neuroimmunology, Department of Neurology, University of Michigan School of Medicine, Ann Arbor, MI 48109 Edited by Ben A. Barres, Stanford University School of Medicine, Stanford, CA, and approved January 22, 2015 (received for review December 22, 2014) Innate immunity can facilitate nervous system regeneration, yet growth can be undermined by concurrent toxicity (9). A deeper the underlying cellular and molecular mechanisms are not well understanding of these opposing effects will be important for understood. Here we show that intraocular injection of lipopoly- exploiting immunomodulatory pathways to promote neural re- saccharide (LPS), a bacterial cell wall component, or the fungal cell pair while minimizing bystander damage. wall extract zymosan both lead to rapid and comparable intravi- In the present study, we investigated the pathways that drive treal accumulation of blood-derived myeloid cells. However, when innate immune-mediated axon regeneration after ONC. We in- combined with retro-orbital optic nerve crush injury, lengthy duced sterile inflammation in the vitreous on the day of injury by growth of severed retinal ganglion cell (RGC) axons occurs only i.o. administration of zymosan or constituents of zymosan clas- in zymosan-injected mice, and not in LPS-injected mice. In mice sified as pathogen-associated molecular patterns (PAMPs). PAMPs dectin-1 deficient for the pattern recognition receptor but not are highly conserved microbial structures that serve as ligands for TLR2 , Toll-like receptor-2 ( ) zymosan-mediated RGC regeneration pattern recognition receptors (PRRs). -
Activated Peripheral-Blood-Derived Mononuclear Cells
Transcription factor expression in lipopolysaccharide- activated peripheral-blood-derived mononuclear cells Jared C. Roach*†, Kelly D. Smith*‡, Katie L. Strobe*, Stephanie M. Nissen*, Christian D. Haudenschild§, Daixing Zhou§, Thomas J. Vasicek¶, G. A. Heldʈ, Gustavo A. Stolovitzkyʈ, Leroy E. Hood*†, and Alan Aderem* *Institute for Systems Biology, 1441 North 34th Street, Seattle, WA 98103; ‡Department of Pathology, University of Washington, Seattle, WA 98195; §Illumina, 25861 Industrial Boulevard, Hayward, CA 94545; ¶Medtronic, 710 Medtronic Parkway, Minneapolis, MN 55432; and ʈIBM Computational Biology Center, P.O. Box 218, Yorktown Heights, NY 10598 Contributed by Leroy E. Hood, August 21, 2007 (sent for review January 7, 2007) Transcription factors play a key role in integrating and modulating system. In this model system, we activated peripheral-blood-derived biological information. In this study, we comprehensively measured mononuclear cells, which can be loosely termed ‘‘macrophages,’’ the changing abundances of mRNAs over a time course of activation with lipopolysaccharide (LPS). We focused on the precise mea- of human peripheral-blood-derived mononuclear cells (‘‘macro- surement of mRNA concentrations. There is currently no high- phages’’) with lipopolysaccharide. Global and dynamic analysis of throughput technology that can precisely and sensitively measure all transcription factors in response to a physiological stimulus has yet to mRNAs in a system, although such technologies are likely to be be achieved in a human system, and our efforts significantly available in the near future. To demonstrate the potential utility of advanced this goal. We used multiple global high-throughput tech- such technologies, and to motivate their development and encour- nologies for measuring mRNA levels, including massively parallel age their use, we produced data from a combination of two distinct signature sequencing and GeneChip microarrays. -
Feedback Regulation Between Initiation and Maturation Networks Orchestrates the Chromatin Dynamics of Epidermal Lineage
bioRxiv preprint doi: https://doi.org/10.1101/349308; this version posted June 18, 2018. The copyright holder for this preprint (which was not certified by peer review) is the author/funder, who has granted bioRxiv a license to display the preprint in perpetuity. It is made available under aCC-BY-NC-ND 4.0 International license. Li et al., p. 1 Feedback Regulation between Initiation and Maturation Networks Orchestrates the Chromatin Dynamics of Epidermal Lineage Commitment Lingjie Li1,3,4, Yong Wang2,4,7,8*, Jessica L. Torkelson1,3*, Gautam Shankar1, Jillian M. Pattison1,3, Hanson H. Zhen1,3, Zhana Duren2,4,7, Fengqin Fang5, Sandra P. Melo1, Samantha N. Piekos1,3, Jiang Li1, Eric J. Liaw1, Lang Chen7, Rui Li1,4, Marius Wernig6, Wing H. Wong2,4, Howard Y. Chang1,4, Anthony E. Oro1,3,9 1 Program in Epithelial Biology and Department of Dermatology 2 Department of Statistics and Biomedical Data Science 3 Center for Definitive and Curative Medicine 4 Center for Personal Dynamic Regulome 5 Division of Immunology and Rheumatology, Department of Medicine, 6 Institute for Stem Cell Biology and Regenerative Medicine, Department of Pathology, Stanford University School of Medicine, Stanford, CA 94305, USA. 7 CEMS, NCMIS, MDIS, Academy of Mathematics & Systems Science, Chinese Academy of Sciences, Beijing,100080, China 8 Center for Excellence in Animal Evolution and Genetics, Chinese Academy of Sciences, Kunming, 650223, China *These authors made equal and independent contributions. 9 Correspondence to Lead Contact: Anthony E. Oro at [email protected] bioRxiv preprint doi: https://doi.org/10.1101/349308; this version posted June 18, 2018. -
CDC2 Mediates Progestin Initiated Endometrial Stromal Cell Proliferation: a PR Signaling to Gene Expression Independently of Its Binding to Chromatin
CDC2 Mediates Progestin Initiated Endometrial Stromal Cell Proliferation: A PR Signaling to Gene Expression Independently of Its Binding to Chromatin Griselda Vallejo1, Alejandro D. La Greca1., Inti C. Tarifa-Reischle1., Ana C. Mestre-Citrinovitz1, Cecilia Ballare´ 2, Miguel Beato2,3, Patricia Saragu¨ eta1* 1 Instituto de Biologı´a y Medicina Experimental, IByME-Conicet, Buenos Aires, Argentina, 2 Centre de Regulacio´ Geno`mica, (CRG), Barcelona, Spain, 3 University Pompeu Fabra (UPF), Barcelona, Spain Abstract Although non-genomic steroid receptor pathways have been studied over the past decade, little is known about the direct gene expression changes that take place as a consequence of their activation. Progesterone controls proliferation of rat endometrial stromal cells during the peri-implantation phase of pregnancy. We showed that picomolar concentration of progestin R5020 mimics this control in UIII endometrial stromal cells via ERK1-2 and AKT activation mediated by interaction of Progesterone Receptor (PR) with Estrogen Receptor beta (ERb) and without transcriptional activity of endogenous PR and ER. Here we identify early downstream targets of cytoplasmic PR signaling and their possible role in endometrial stromal cell proliferation. Microarray analysis of global gene expression changes in UIII cells treated for 45 min with progestin identified 97 up- and 341 down-regulated genes. The most over-represented molecular functions were transcription factors and regulatory factors associated with cell proliferation and cell cycle, a large fraction of which were repressors down-regulated by hormone. Further analysis verified that progestins regulate Ccnd1, JunD, Usf1, Gfi1, Cyr61, and Cdkn1b through PR- mediated activation of ligand-free ER, ERK1-2 or AKT, in the absence of genomic PR binding. -
Genome-Wide DNA Methylation Analysis of KRAS Mutant Cell Lines Ben Yi Tew1,5, Joel K
www.nature.com/scientificreports OPEN Genome-wide DNA methylation analysis of KRAS mutant cell lines Ben Yi Tew1,5, Joel K. Durand2,5, Kirsten L. Bryant2, Tikvah K. Hayes2, Sen Peng3, Nhan L. Tran4, Gerald C. Gooden1, David N. Buckley1, Channing J. Der2, Albert S. Baldwin2 ✉ & Bodour Salhia1 ✉ Oncogenic RAS mutations are associated with DNA methylation changes that alter gene expression to drive cancer. Recent studies suggest that DNA methylation changes may be stochastic in nature, while other groups propose distinct signaling pathways responsible for aberrant methylation. Better understanding of DNA methylation events associated with oncogenic KRAS expression could enhance therapeutic approaches. Here we analyzed the basal CpG methylation of 11 KRAS-mutant and dependent pancreatic cancer cell lines and observed strikingly similar methylation patterns. KRAS knockdown resulted in unique methylation changes with limited overlap between each cell line. In KRAS-mutant Pa16C pancreatic cancer cells, while KRAS knockdown resulted in over 8,000 diferentially methylated (DM) CpGs, treatment with the ERK1/2-selective inhibitor SCH772984 showed less than 40 DM CpGs, suggesting that ERK is not a broadly active driver of KRAS-associated DNA methylation. KRAS G12V overexpression in an isogenic lung model reveals >50,600 DM CpGs compared to non-transformed controls. In lung and pancreatic cells, gene ontology analyses of DM promoters show an enrichment for genes involved in diferentiation and development. Taken all together, KRAS-mediated DNA methylation are stochastic and independent of canonical downstream efector signaling. These epigenetically altered genes associated with KRAS expression could represent potential therapeutic targets in KRAS-driven cancer. Activating KRAS mutations can be found in nearly 25 percent of all cancers1. -
Ten Commandments for a Good Scientist
Unravelling the mechanism of differential biological responses induced by food-borne xeno- and phyto-estrogenic compounds Ana María Sotoca Covaleda Wageningen 2010 Thesis committee Thesis supervisors Prof. dr. ir. Ivonne M.C.M. Rietjens Professor of Toxicology Wageningen University Prof. dr. Albertinka J. Murk Personal chair at the sub-department of Toxicology Wageningen University Thesis co-supervisor Dr. ir. Jacques J.M. Vervoort Associate professor at the Laboratory of Biochemistry Wageningen University Other members Prof. dr. Michael R. Muller, Wageningen University Prof. dr. ir. Huub F.J. Savelkoul, Wageningen University Prof. dr. Everardus J. van Zoelen, Radboud University Nijmegen Dr. ir. Toine F.H. Bovee, RIKILT, Wageningen This research was conducted under the auspices of the Graduate School VLAG Unravelling the mechanism of differential biological responses induced by food-borne xeno- and phyto-estrogenic compounds Ana María Sotoca Covaleda Thesis submitted in fulfillment of the requirements for the degree of doctor at Wageningen University by the authority of the Rector Magnificus Prof. dr. M.J. Kropff, in the presence of the Thesis Committee appointed by the Academic Board to be defended in public on Tuesday 14 September 2010 at 4 p.m. in the Aula Unravelling the mechanism of differential biological responses induced by food-borne xeno- and phyto-estrogenic compounds. Ana María Sotoca Covaleda Thesis Wageningen University, Wageningen, The Netherlands, 2010, With references, and with summary in Dutch. ISBN: 978-90-8585-707-5 “Caminante no hay camino, se hace camino al andar. Al andar se hace camino, y al volver la vista atrás se ve la senda que nunca se ha de volver a pisar” - Antonio Machado – A mi madre. -
Genome-Wide Assessment of REST Binding Profiles Reveals Distinctions Between Human and Mouse Hippocampus
bioRxiv preprint doi: https://doi.org/10.1101/2020.07.07.192229; this version posted July 7, 2020. The copyright holder for this preprint (which was not certified by peer review) is the author/funder, who has granted bioRxiv a license to display the preprint in perpetuity. It is made available under aCC-BY-NC-ND 4.0 International license. Genome-wide assessment of REST binding profiles reveals distinctions between human and mouse hippocampus James C. McGann1,2#, Michael Spinner1#, Saurabh K. Garg1,3#, Karin Mullendorf1,4, Randall L. Woltjer5 and Gail Mandel1*. 1 Vollum Institute, Oregon Health and Science University, Portland, OR, USA. 2 Cancer Early Detection Advanced Research Center, Oregon Health and Science Institute, Portland, OR, USA. 3 Department of Cutaneous Oncology, H. Lee Moffitt Cancer Center and Research Institute, Tampa, FL, USA. 4 Universidad San Sebastian, Puerto Montt, Chile 5 Department of Pathology, Division of Neuropathology, Oregon Health and Science University, Portland, OR, USA. *Corresponding author # These authors contributed equally Abstract Background The transcriptional repressor, RE1 Silencing Transcription Factor (REST), recognized historically as a master regulator of neuronal gene expression during mouse development, has recently been ascribed roles in human aging and neurodegenerative disorders. However, REST’s role in healthy adult human brain, and how faithfully mouse models reproduce REST function in human brain, is not known. Results Here, we present the first genome-wide binding profile for REST in both mouse and human postnatal hippocampus. We find the majority of REST-bound sites in human hippocampus are unique compared to both mouse hippocampus and to all other reported human ENCODE cell types. -
The Unfolded Protein Response: an Overview
biology Review The Unfolded Protein Response: An Overview Adam Read 1,2 and Martin Schröder 1,2,* 1 Department of Biosciences, Durham University, South Road, Durham DH1 3LE, UK; [email protected] 2 Biophysical Sciences Institute, Durham University, South Road, Durham DH1 3LE, UK * Correspondence: [email protected]; Tel.: +44-191-334-1316 Simple Summary: The unfolded protein response (UPR) is the cells’ way of maintaining the balance of protein folding in the endoplasmic reticulum, which is the section of the cell designated for folding proteins with specific destinations such as other organelles or to be secreted by the cell. The UPR is activated when unfolded proteins accumulate in the endoplasmic reticulum. This accumulation puts a greater load on the molecules in charge of folding the proteins, and therefore the UPR works to balance this by lowering the number of unfolded proteins present in the cell. This is done in multiple ways, such as lowering the number of proteins that need to be folded; increasing the folding ability of the endoplasmic reticulum and by removing some of the unfolded proteins which take longer to fold. If the UPR is successful at reducing the number of unfolded proteins, the UPR is inactivated and the cells protein folding balance is returned to normal. However, if the UPR is unsuccessful, then this can lead to cell death. Abstract: The unfolded protein response is the mechanism by which cells control endoplasmic reticulum (ER) protein homeostasis. Under normal conditions, the UPR is not activated; however, under certain stresses, such as hypoxia or altered glycosylation, the UPR can be activated due to an accumulation of unfolded proteins. -
Combinatorial Bzip Dimers Display Complex DNA-Binding Specificity Landscapes
Combinatorial bZIP dimers display complex DNA-binding specificity landscapes The MIT Faculty has made this article openly available. Please share how this access benefits you. Your story matters. Citation Rodriguez-Martinez, Jose A et al. “Combinatorial bZIP Dimers Display Complex DNA-Binding Specificity Landscapes.” eLife 6 (2017): n. pag. As Published http://dx.doi.org/10.7554/eLife.19272 Publisher eLife Sciences Publications, Ltd. Version Final published version Citable link http://hdl.handle.net/1721.1/110147 Terms of Use Creative Commons Attribution 4.0 International License Detailed Terms http://creativecommons.org/licenses/by-nc/4.0/ RESEARCH ARTICLE Combinatorial bZIP dimers display complex DNA-binding specificity landscapes Jose´ A Rodrı´guez-Martı´nez1†, Aaron W Reinke2†, Devesh Bhimsaria1,3†, Amy E Keating2,4, Aseem Z Ansari1,5* 1Department of Biochemistry, University of Wisconsin-Madison, Madison, United States; 2Department of Biology, Massachusetts Institute of Technology, Cambridge, United States; 3Department of Electrical and Computer Engineering, University of Wisconsin-Madison, Madison, Unites States; 4Department of Biological Engineering, Massachusetts Institute of Technology, Cambridge, United States; 5The Genome Center of Wisconsin, University of Wisconsin-Madison, Madison, United States Abstract How transcription factor dimerization impacts DNA-binding specificity is poorly understood. Guided by protein dimerization properties, we examined DNA binding specificities of 270 human bZIP pairs. DNA interactomes of 80 heterodimers and 22 homodimers revealed that 72% of heterodimer motifs correspond to conjoined half-sites preferred by partnering monomers. Remarkably, the remaining motifs are composed of variably-spaced half-sites (12%) or ‘emergent’ sites (16%) that cannot be readily inferred from half-site preferences of partnering monomers. -
Distinct Roles of Jun : Fos and Jun : ATF Dimers in Oncogenesis
Oncogene (2001) 20, 2453 ± 2464 ã 2001 Nature Publishing Group All rights reserved 0950 ± 9232/01 $15.00 www.nature.com/onc Distinct roles of Jun : Fos and Jun : ATF dimers in oncogenesis Hans van Dam*,1 and Marc Castellazzi2 1Department of Molecular Cell Biology, Leiden University Medical Center, Sylvius Laboratories, PO Box 9503, 2300 RA Leiden, The Netherlands; 2Unite de Virologie Humaine, Institut National de la Sante et de la Recherche MeÂdicale (INSERM-U412), Ecole Normale SupeÂrieure, 46 alleÂe d'Italie, 69364 Lyon Cedex 07, France Jun : Fos and Jun : ATF complexes represent two classes dimers with emphasis on their roles in oncogenic of AP-1 dimers that (1) preferentially bind to either transformation in avian model systems. Previous heptameric or octameric AP-1 binding sites, and (2) are reviews on AP-1 and cell transformation include dierently regulated by cellular signaling pathways and references: (Angel and Karin, 1991; Wisdom, 1999; oncogene products. To discriminate between the func- Vogt, 1994; Karin et al., 1997; van Dam and van der tions of Jun : Fos, Jun: ATF and Jun : Jun, mutants were Eb, 1994; Hagmeyer et al., 1995). developed that restrict the ability of Jun to dimerize either to itself, or to Fos(-like) or ATF(-like) partners. Introduction of these mutants in chicken embryo Jun : Fos and Jun : ATF transcription factors: dimeric ®broblasts shows that Jun : Fra2 and Jun : ATF2 dimers complexes with variable composition and activities play distinct, complementary roles in in vitro oncogenesis by inducing either anchorage independence or growth AP-1 sub-units: members of the bZip protein family factor independence, respectively. -
Tgfβ-Regulated Gene Expression by Smads and Sp1/KLF-Like Transcription Factors in Cancer VOLKER ELLENRIEDER
ANTICANCER RESEARCH 28 : 1531-1540 (2008) Review TGFβ-regulated Gene Expression by Smads and Sp1/KLF-like Transcription Factors in Cancer VOLKER ELLENRIEDER Signal Transduction Laboratory, Internal Medicine, Department of Gastroenterology and Endocrinology, University of Marburg, Marburg, Germany Abstract. Transforming growth factor beta (TGF β) controls complex induces the canonical Smad signaling molecules which vital cellular functions through its ability to regulate gene then translocate into the nucleus to regulate transcription (2). The expression. TGFβ binding to its transmembrane receptor cellular response to TGF β can be extremely variable depending kinases initiates distinct intracellular signalling cascades on the cell type and the activation status of a cell at a given time. including the Smad signalling and transcription factors and also For instance, TGF β induces growth arrest and apoptosis in Smad-independent pathways. In normal epithelial cells, TGF β healthy epithelial cells, whereas it can also promote tumor stimulation induces a cytostatic program which includes the progression through stimulation of cell proliferation and the transcriptional repression of the c-Myc oncogene and the later induction of an epithelial-to-mesenchymal transition of tumor induction of the cell cycle inhibitors p15 INK4b and p21 Cip1 . cells (1, 3). In the last decade it has become clear that both the During carcinogenesis, however, many tumor cells lose their tumor suppressing and the tumor promoting functions of TGF β ability to respond to TGF β with growth inhibition, and instead, are primarily regulated on the level of gene expression through activate genes involved in cell proliferation, invasion and Smad-dependent and -independent mechanisms (1, 2, 4).