Armillaria Root Rot of Peach
Total Page:16
File Type:pdf, Size:1020Kb
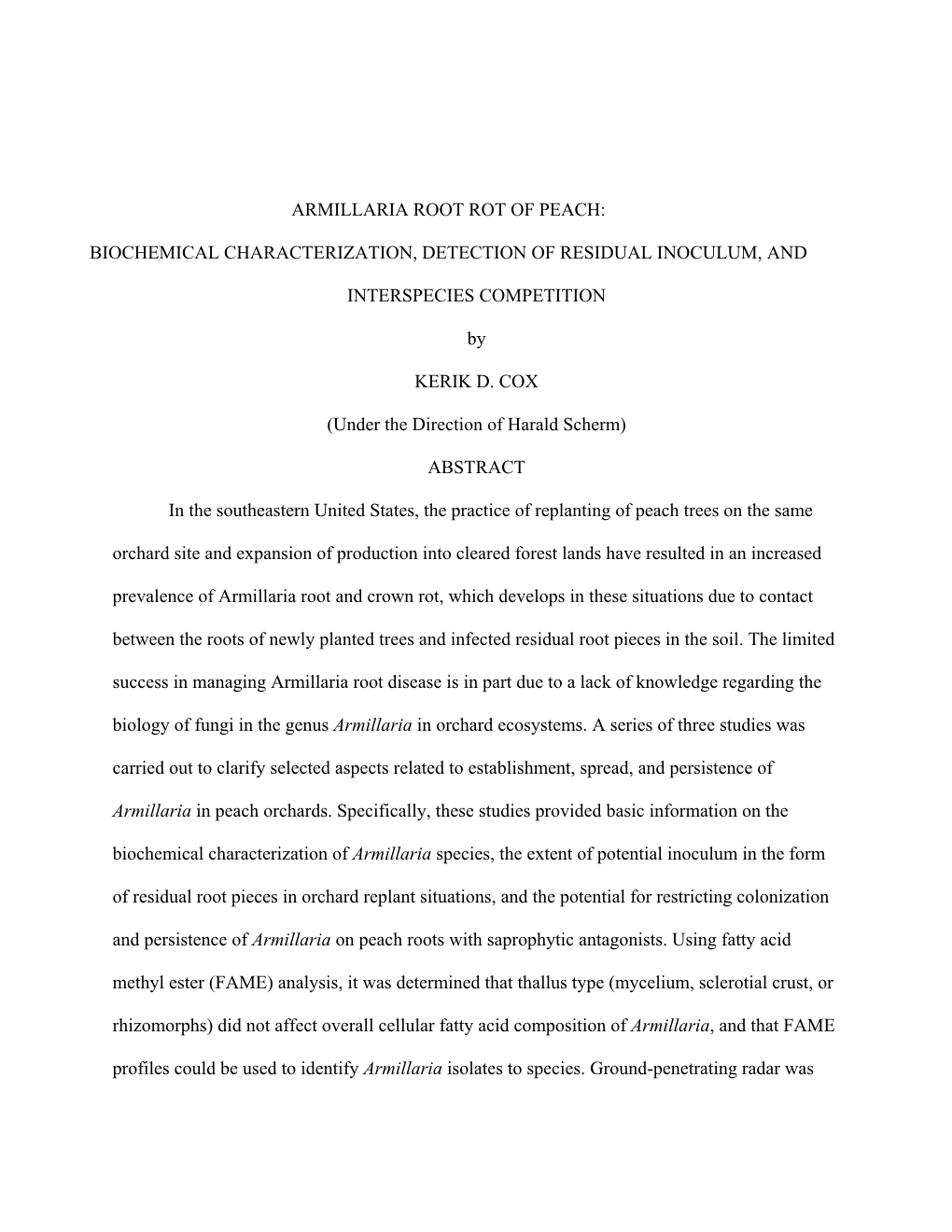
Load more
Recommended publications
-
<I>Hydropus Mediterraneus</I>
ISSN (print) 0093-4666 © 2012. Mycotaxon, Ltd. ISSN (online) 2154-8889 MYCOTAXON http://dx.doi.org/10.5248/121.393 Volume 121, pp. 393–403 July–September 2012 Laccariopsis, a new genus for Hydropus mediterraneus (Basidiomycota, Agaricales) Alfredo Vizzini*, Enrico Ercole & Samuele Voyron Dipartimento di Scienze della Vita e Biologia dei Sistemi - Università degli Studi di Torino, Viale Mattioli 25, I-10125, Torino, Italy *Correspondence to: [email protected] Abstract — Laccariopsis (Agaricales) is a new monotypic genus established for Hydropus mediterraneus, an arenicolous species earlier often placed in Flammulina, Oudemansiella, or Xerula. Laccariopsis is morphologically close to these genera but distinguished by a unique combination of features: a Laccaria-like habit (distant, thick, subdecurrent lamellae), viscid pileus and upper stipe, glabrous stipe with a long pseudorhiza connecting with Ammophila and Juniperus roots and incorporating plant debris and sand particles, pileipellis consisting of a loose ixohymeniderm with slender pileocystidia, large and thin- to thick-walled spores and basidia, thin- to slightly thick-walled hymenial cystidia and caulocystidia, and monomitic stipe tissue. Phylogenetic analyses based on a combined ITS-LSU sequence dataset place Laccariopsis close to Gloiocephala and Rhizomarasmius. Key words — Agaricomycetes, Physalacriaceae, /gloiocephala clade, phylogeny, taxonomy Introduction Hydropus mediterraneus was originally described by Pacioni & Lalli (1985) based on collections from Mediterranean dune ecosystems in Central Italy, Sardinia, and Tunisia. Previous collections were misidentified as Laccaria maritima (Theodor.) Singer ex Huhtinen (Dal Savio 1984) due to their laccarioid habit. The generic attribution to Hydropus Kühner ex Singer by Pacioni & Lalli (1985) was due mainly to the presence of reddish watery droplets on young lamellae and sarcodimitic tissue in the stipe (Corner 1966, Singer 1982). -
SOMA News March 2011
VOLUME 23 ISSUE 7 March 2011 SOMA IS AN EDUCATIONAL ORGANIZATION DEDICATED TO MYCOLOGY. WE ENCOURAGE ENVIRONMENTAL AWARENESS BY SHARING OUR ENTHUSIASM THROUGH PUBLIC PARTICIPATION AND GUIDED FORAYS. WINTER/SPRING 2011 SPEAKER OF THE MONTH SEASON CALENDAR March Connie and Patrick March 17th » Meeting—7pm —“A Show and Tell”— Sonoma County Farm Bureau Speaker: Connie Green & Patrick March 17th—7pm Hamilton Foray March. 19th » Salt Point April April 21st » Meeting—7pm Sonoma County Farm Bureau Speaker: Langdon Cook Foray April 23rd » Salt Point May May 19th » Meeting—7pm Sonoma County Farm Bureau Speaker: Bob Cummings Foray May: Possible Morel Camping! eparated at birth but from the same litter Connie Green and Patrick Hamilton have S traveled (endured?) mushroom journeys together for almost two decades. They’ve been to the humid and hot jaguar jungles of Chiapas chasing tropical mushrooms and to EMERGENCY the cloud forests of the Sierra Madre for boletes and Indigo milky caps. In the cold and wet wilds of Alaska they hiked a spruce and hemlock forest trail to watch grizzly bears MUSHROOM tearing salmon bellies just a few yards away. POISONING IDENTIFICATION In the remote Queen Charlotte Islands their bush plane flew over “fields of golden chanterelles,” landed on the ocean, and then off into a zany Zodiac for a ride over a cold After seeking medical attention, contact and roiling sea alongside some low flying puffins to the World Heritage Site of Ninstints. Darvin DeShazer for identification at The two of them have gazed at glaciers and berry picked on muskeg bogs. More than a (707) 829-0596. -
Biological Control of Rhizoctonia Solani by Indigenous Trichoderma Spp
Hebron University Research Journal. H.U.R.J. is available online at Vol.(3), No.(1), pp.(1 – 15), 2007 http://www.hebron.edu/journal Biological Control of Rhizoctonia solani by Indigenous Trichoderma spp. Isolates from Palestine 1 1 *Radwan M. Barakat , Fadel Al-Mahareeq , 2 1 Mohammed S. Ali -Shtayeh , and Mohammad I. AL- Masri 1Faculty of Agriculture, Hebron University, Hebron- Palestine 2Faculty of Science, An-Najah National University, Nablus- Palestine Abstract: The effect of indigenous Trichoderma isolates against the soil-borne phytopathogen Rhizoctonia solani was investigated in dual culture and bioassay on bean plants. Ap- plication of the bioagent isolates as a conidial suspension (3*107) greatly reduced the disease index of bean plants caused by R. solani in different rates and the most effective Trichoderma harzianum isolate (Jn14) reduced the disease by 65%. In dual culture, the T. harzianum (Jn14) overgrew the pathogen R. solani in an average of 16.75 mm/day at 30 °C. In addition, the results showed that T. harzianum (Jn14) and T. hamatum (T36) were the most effective isolates at 25°C and inhibited R. solani mycelial growth by 42% and 78% respectively, due to fungitoxic metabolites production. The Effect of Trichoderma on bean seedlings growth was obvious; height was nearly doubled (160% - 200%), while fresh and dry weights increased by 133% and 217%, respectively. Ger- mination of bean seeds in treated soil with Trichoderma isolates occurred about four days earlier than those in untreated soils. The results revealed however some variation between isolates which was due to genetic variation, mycelium-coiling rate, sporulation rate, fungitoxic metabolites, induced growth response and temperature effect. -
Shoestring Root Rot - a Cause of Tree and Shrub Decline
PPFS-OR-W-05 Plant Pathology Fact Sheet Shoestring Root Rot - A Cause of Tree and Shrub Decline By John Hartman Most woody landscape plants are susceptible to shoestring root rot, cause of dieback and decline in the landscape. Diagnosis of this problem requires close examination of the base of the trunk which often reveals loose or decayed bark and dead cambium. By peeling back the bark one can often observe dark brown rhizomorphs (thick strands of hyphae), resembling narrow “shoestrings” (Figure 1). FIGURE 1. ARMILLARIA RHIZOMORPHS These rhizomorphs are signs of one or more species of Armillaria and perhaps other This disease is sometimes confused with related fungi, cause of shoestring root rot. Phytophthora root and collar rot. The decay Decayed roots with rhizomorphs growing associated with Phytophthora is usually a along their surface can be observed by darker brown color, decayed bark is more digging up the roots. moist, and rhizomorphs are absent. Creamy white fans of fungal mycelium Shoestring root rot has a very wide host range may also be observed under the bark. The and is most frequently observed in oaks, mushroom stage (Figure 2) of shoestring maples, pines, hornbeams, taxus, and fruit root rot sometimes develops in fall on dead trees in the landscape. Often, it is associated trees or decayed logs. with formerly wooded sites converted to landscapes because the fungus can persist Shoestring root rot is also called Armillaria for many years in decaying wood in soil. root rot, mushroom root rot, and oak root rot. Most trees with Armillaria root rot are trees Once trees or shrubs begin to show serious symptoms of decline, including dieback of twigs and branches, undersized and off-color leaves, increased trunk and limb sprouts (epicormic branching), and excessive fruit set, the decline is often not reversible. -
Root Diseases Diagnosis of Root Diseases Can Be Very Challenging Armillaria Root Disease • Symptoms Can Be Similar for Different Root Diseases • Below Ground Attacks
Root Diseases Diagnosis of root diseases can be very challenging Armillaria root disease • Symptoms can be similar for different root diseases • Below ground attacks but above ground Heterobasision annosum symptoms Annosus root disease • Signs are rare and often are produced once a year for short periods Chronosequence of stand and tree level symptoms of root diseases Source: Fig.12.10, p.312 Forest Health and Protection by Edmonds, R. L., J. K. Agee and R. I. Gara. 2011. Waveland Press, Long Grove, IL. 2nd ed. Used with permission from Waveland Press Dec.13, 2011. Tree level symptoms of Armillaria root disease CC BY 3.0. Borys M. Tkacz, USFS, Bugwood.orgTkacz, BorysM. 3.0. CC BY Tree level symptoms of Armillaria root disease • Dead saplings next to stumps, retaining needles • Roots of young trees grow into the dead roots infected by Armillaria, come into contact with rhizomorphs and get infected Signs of Armillaria root disease • Rhizomorphs: specialized highly adapted structures • Allow the pathogen to explore the “Rhizomorphs (thick fungal threads) of Armillaria mellea” Lairich Rig. CC BY-SA 2.0. environment and http://www.geograph.org.uk/photo/933530 survive in the soil for decades • Contain melanin, a protective compound Cross section of rhizomorph showing differentiated tissue Signs of Armillaria root disease • Armillaria attacks the living cambium of tree roots • Mycelial fans form under the bark of infected trees • The mycelium is very strong and can grow under and lift the bark, leaving imprints Signs of Armillaria root disease -
Checklist of Macro-Fungi from Baramati Area of Pune District, MS, India
Int.J.Curr.Microbiol.App.Sci (2019) 8(7): 2187-2192 International Journal of Current Microbiology and Applied Sciences ISSN: 2319-7706 Volume 8 Number 07 (2019) Journal homepage: http://www.ijcmas.com Original Research Article https://doi.org/10.20546/ijcmas.2019.807.265 Checklist of Macro-Fungi from Baramati Area of Pune District, MS, India Anuradha K. Bhosale*, Vivek Kadam, Prasad Bankar, Sandhya Shitole, Sourabh Chandankar, Sujit Wagh and M.B. Kanade P. G. Research Center, Department of Botany, Tuljaram Chaturchand College of Arts, Science and Commerce, Baramati, Dist. Pune - 413 102, Maharashtra, India *Corresponding author ABSTRACT Macro-fungi are the fungal species that produce fruiting bodies visible to naked eyes and occurs widely in the rainy season. The macro-fungi plays K e yw or ds important role in nutrient dynamics, soil health, as pollution indicator, Macro-fungi species mutualism and its interaction and even has its economic role in diversity carbon cycling and the mobilization of nitrogen and phosphorous. Present investigation emphasizes on study of macro-fungi from Baramati area of Article Info Pune district of Maharashtra. During the study frequent field visits, listing Accepted: of genera and their species, identification and photography has done. In the 17 June 2019 Available Online: checklist total 64 fungal species belonging to 37 genera, 03 sub-divisions, 10 July 2019 13 orders and 23 families were reported. The contribution of Basidiomycotina fungi was 90% followed by Ascomycotina (7.8%) and Zygomycotina (1.6%). Introduction than 27000 fungal species throughout the India. The number of mushroom species Fungi are amongst the most important alone, recorded in the world were 41,000 of organisms in the world, not only because of which approximately 850 species were their vital role in ecosystem functions recorded from India (Deshmukh, 2004) mostly (Blackwell, 2011) but also for their influence belonging to gilled mushrooms. -
„Behandlung Von Textilfarbstoffen Durch Aquatische Pilze“ D
„Behandlung von Textilfarbstoffen durch aquatische Pilze“ D i s s e r t a t i o n zur Erlangung des akademischen Grades doctor rerum naturalium (Dr. rer. nat.) vorgelegt der Naturwissenschaftlichen Fakultät I Biowissenschaften der Martin-Luther-Universität Halle-Wittenberg in Zusammenarbeit mit dem Lehrstuhl für Umweltbiotechnologie des Internationalen Hochschulinstitutes Zittau von Herrn Diplom-Biologen Charles Junghanns geb. am 09. April 1979 in Leipzig Gutachter 1. Prof. Dr. Gerd-Joachim Krauß 2. Prof. Dr. Martin Hofrichter 3. Prof. Dr. Georg Gübitz Halle (Saale), 05. Juni 2008 urn:nbn:de:gbv:3-000013693 [http://nbn-resolving.de/urn/resolver.pl?urn=nbn%3Ade%3Agbv%3A3-000013693] Inhaltsverzeichnis Inhaltsverzeichnis ABKÜRZUNGSVERZEICHNIS.........................................................................................1 1. EINLEITUNG.......................................................................................................................4 1.1. Aquatische Pilze..........................................................................................................4 1.2. Laccasen (E.C. 1.10.3.2) .............................................................................................8 1.3. Textilfarbstoffe..........................................................................................................10 1.4. Anliegen der Arbeit ..................................................................................................19 2. MATERIAL UND METHODEN......................................................................................20 -
Two New Species and a New Chinese Record of Hypocreaceae As Evidenced by Morphological and Molecular Data
MYCOBIOLOGY 2019, VOL. 47, NO. 3, 280–291 https://doi.org/10.1080/12298093.2019.1641062 RESEARCH ARTICLE Two New Species and a New Chinese Record of Hypocreaceae as Evidenced by Morphological and Molecular Data Zhao Qing Zeng and Wen Ying Zhuang State Key Laboratory of Mycology, Institute of Microbiology, Chinese Academy of Sciences, Beijing, P.R. China ABSTRACT ARTICLE HISTORY To explore species diversity of Hypocreaceae, collections from Guangdong, Hubei, and Tibet Received 13 February 2019 of China were examined and two new species and a new Chinese record were discovered. Revised 27 June 2019 Morphological characteristics and DNA sequence analyses of the ITS, LSU, EF-1a, and RPB2 Accepted 4 July 2019 regions support their placements in Hypocreaceae and the establishments of the new spe- Hypomyces hubeiensis Agaricus KEYWORDS cies. sp. nov. is characterized by occurrence on fruitbody of Hypomyces hubeiensis; sp., concentric rings formed on MEA medium, verticillium-like conidiophores, subulate phia- morphology; phylogeny; lides, rod-shaped to narrowly ellipsoidal conidia, and absence of chlamydospores. Trichoderma subiculoides Trichoderma subiculoides sp. nov. is distinguished by effuse to confluent rudimentary stro- mata lacking of a well-developed flank and not changing color in KOH, subcylindrical asci containing eight ascospores that disarticulate into 16 dimorphic part-ascospores, verticillium- like conidiophores, subcylindrical phialides, and subellipsoidal to rod-shaped conidia. Morphological distinctions between the new species and their close relatives are discussed. Hypomyces orthosporus is found for the first time from China. 1. Introduction Members of the genus are mainly distributed in temperate and tropical regions and economically The family Hypocreaceae typified by Hypocrea Fr. -
Mushroom Toxins & Poisonings in New Jersey
Mushroom Toxins & Poisonings in New Jersey & Nearby Eastern North America What this document doesn’t do: (1) This document is not intended to be used as a guide for treatment and should not be so used. (2) Mushrooms should not be selected for eating based on the content of this document. [In identifying mushrooms in poisoning cases, this document does not replace expertise that should be obtained by calling NJPIES and obtaining contact with an experienced mycologist.] (3) This document is not a replacement for a detailed toxicological review of the subject of mushroom poisoning. (4) This document is intended for use with a broad set of audiences; for this reasons, it should not be used uncritically in setting protocols [for example, carrying out a Meixner test would be inappropriate for a first responder who would appropriately focus on collecting a poi- soning victim, the relevant objects from the scene of the poisoning, and the critical timing characteristics of the event such as the delay between ingestion and onset of symptoms.] POISON CONTROL: New Jersey “Poison Control” is called NJPIES (New Jersey Poison Information & Education System). Telephone: 1-800-222-1222 [works in all states—(WARN- ING) WILL CONNECT TO A MOBILE PHONE’S HOME STATE—IF YOU’RE UNCERTAIN, USE A LAND- LINE] If the victim is unconscious, call “911.” Background of these notes: This document was originally compiled by Rod Tulloss and Dorothy Smullen for an NJ Mycol. Assoc. workshop, 25 March 2006. Version 2.0 was compiled by Tulloss. When viewed with Acrobat Reader, underlined red or gray words and phrases are “hot linked cross-references.” We have included a few notes on fungal poisons that are not from “mushrooms.” The notes were prepared by mycologists with experience in diagnosis of fungi involved in cases in which ingestion of toxic fungi was suspected. -
Phylogenetic Assignment of the Fungicolous Hypoxylon Invadens (Ascomycota, Xylariales) and Investigation of Its Secondary Metabolites
microorganisms Article Phylogenetic Assignment of the Fungicolous Hypoxylon invadens (Ascomycota, Xylariales) and Investigation of its Secondary Metabolites Kevin Becker 1,2 , Christopher Lambert 1,2,3 , Jörg Wieschhaus 1 and Marc Stadler 1,2,* 1 Department of Microbial Drugs, Helmholtz Centre for Infection Research GmbH (HZI), Inhoffenstraße 7, 38124 Braunschweig, Germany; [email protected] (K.B.); [email protected] (C.L.); [email protected] (J.W.) 2 German Centre for Infection Research Association (DZIF), Partner site Hannover-Braunschweig, Inhoffenstraße 7, 38124 Braunschweig, Germany 3 Department for Molecular Cell Biology, Helmholtz Centre for Infection Research GmbH (HZI) Inhoffenstraße 7, 38124 Braunschweig, Germany * Correspondence: [email protected]; Tel.: +49-531-6181-4240; Fax: +49-531-6181-9499 Received: 23 July 2020; Accepted: 8 September 2020; Published: 11 September 2020 Abstract: The ascomycete Hypoxylon invadens was described in 2014 as a fungicolous species growing on a member of its own genus, H. fragiforme, which is considered a rare lifestyle in the Hypoxylaceae. This renders H. invadens an interesting target in our efforts to find new bioactive secondary metabolites from members of the Xylariales. So far, only volatile organic compounds have been reported from H. invadens, but no investigation of non-volatile compounds had been conducted. Furthermore, a phylogenetic assignment following recent trends in fungal taxonomy via a multiple sequence alignment seemed practical. A culture of H. invadens was thus subjected to submerged cultivation to investigate the produced secondary metabolites, followed by isolation via preparative chromatography and subsequent structure elucidation by means of nuclear magnetic resonance (NMR) spectroscopy and high-resolution mass spectrometry (HR-MS). -
ARMILLARIA ROOT ROT: a DISEASE of NATIVE and INTRODUCED TREES Forests Fact Sheet
ARMILLARIA ROOT ROT: A DISEASE December 2003 OF NATIVE AND INTRODUCED TREES ISSN 1440-2262 Ian W. Smith & David I. Smith, Forest Science Centre Background Armillaria root rot, unlike some familiar pests and diseases, occurs naturally in many forests of Victoria. This aggressive fungal pathogen has so far seriously damaged about 2000 ha of mixed species eucalypt forest in the Mt. Cole and Wombat State Forests with minor outbreaks in other areas of Victoria. It attacks many species of native trees and shrubs including eucalypts and wattles, and has also been recorded on introduced trees and shrubs growing in parks and botanical and domestic gardens. Symptoms and Signs of disease The fungus can kill trees and shrub species of any age and has a very wide host range. Young trees and seedlings may, over a period of a few weeks, suddenly wilt and the leaves fall. They may die within 3-6 months of the onset of the first symptoms. Larger trees, especially those with a sizeable trunk, die more slowly. The initial symptoms of sparse foliage and branch dieback may continue for up to 3 years before the remaining foliage begins to wilt, the leaves turn brown and the trees die (Figure 1). Other pathogens and environmental disorders can produce similar symptoms, so it is wise to check that Armillaria infection is present by examining a tree showing advanced disease symptoms. To do this, remove some of the inner bark at the base of the tree and look for fan-shaped, creamy-white fungal sheets (Figure 2). Often the bark tissue around the fungal sheets turns chocolate brown in colour. -
Species List for Arizona Mushroom Society White Mountains Foray August 11-13, 2016
Species List for Arizona Mushroom Society White Mountains Foray August 11-13, 2016 **Agaricus sylvicola grp (woodland Agaricus, possibly A. chionodermus, slight yellowing, no bulb, almond odor) Agaricus semotus Albatrellus ovinus (orange brown frequently cracked cap, white pores) **Albatrellus sp. (smooth gray cap, tiny white pores) **Amanita muscaria supsp. flavivolvata (red cap with yellow warts) **Amanita muscaria var. guessowii aka Amanita chrysoblema (yellow cap with white warts) **Amanita “stannea” (tin cap grisette) **Amanita fulva grp.(tawny grisette, possibly A. “nishidae”) **Amanita gemmata grp. Amanita pantherina multisquamosa **Amanita rubescens grp. (all parts reddening) **Amanita section Amanita (ring and bulb, orange staining volval sac) Amanita section Caesare (prov. name Amanita cochiseana) Amanita section Lepidella (limbatulae) **Amanita section Vaginatae (golden grisette) Amanita umbrinolenta grp. (slender, ringed cap grisette) **Armillaria solidipes (honey mushroom) Artomyces pyxidatus (whitish coral on wood with crown tips) *Ascomycota (tiny, grayish/white granular cups on wood) **Auricularia Americana (wood ear) Auriscalpium vulgare Bisporella citrina (bright yellow cups on wood) Boletus barrowsii (white king bolete) Boletus edulis group Boletus rubriceps (red king bolete) Calyptella capula (white fairy lanterns on wood) **Cantharellus sp. (pink tinge to cap, possibly C. roseocanus) **Catathelesma imperiale Chalciporus piperatus Clavariadelphus ligula Clitocybe flavida aka Lepista flavida **Coltrichia sp. Coprinellus