The Surface Geology and Geomorphology of Phobos
Total Page:16
File Type:pdf, Size:1020Kb
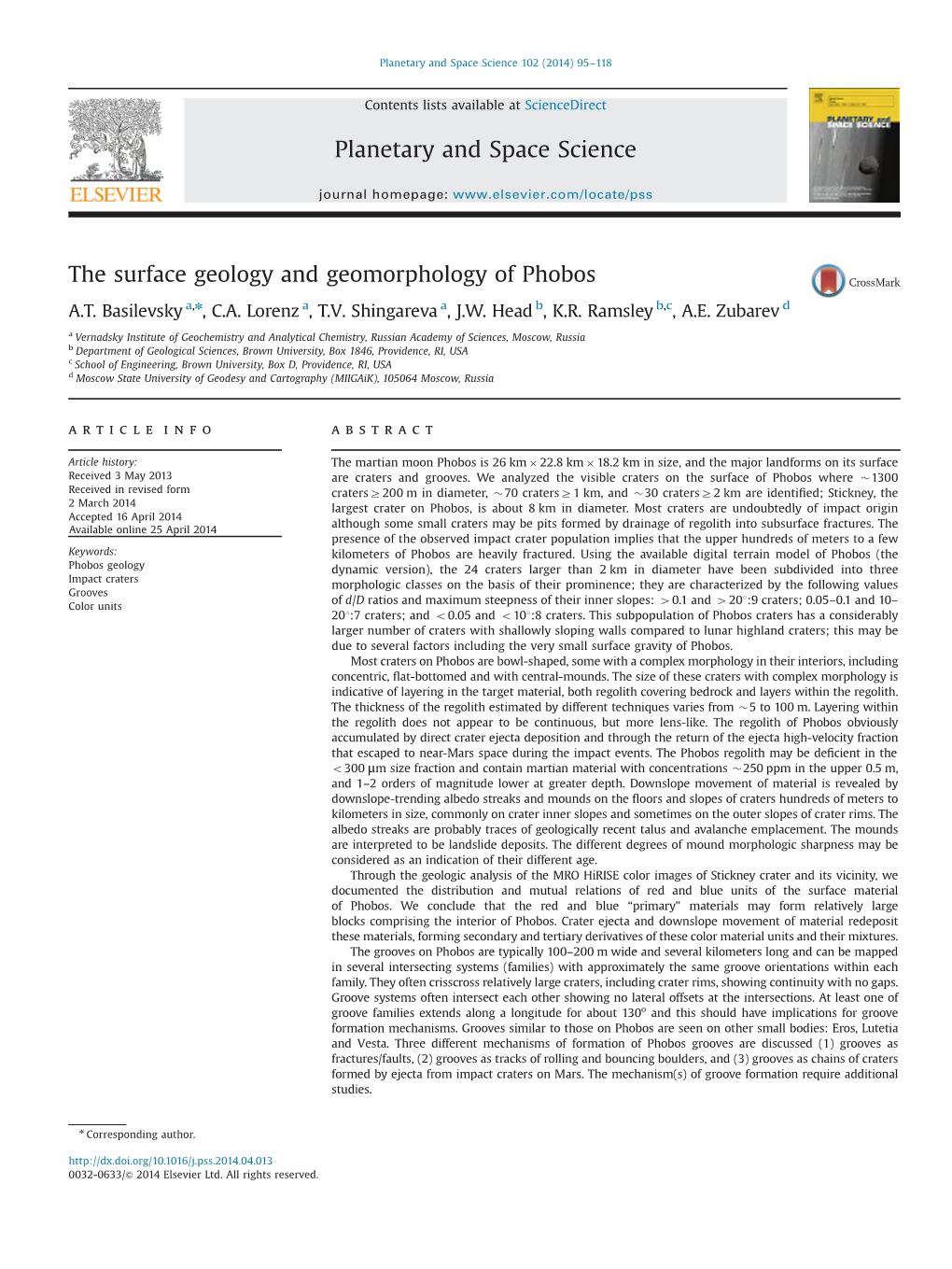
Load more
Recommended publications
-
Phobos, Deimos: Formation and Evolution Alex Soumbatov-Gur
Phobos, Deimos: Formation and Evolution Alex Soumbatov-Gur To cite this version: Alex Soumbatov-Gur. Phobos, Deimos: Formation and Evolution. [Research Report] Karpov institute of physical chemistry. 2019. hal-02147461 HAL Id: hal-02147461 https://hal.archives-ouvertes.fr/hal-02147461 Submitted on 4 Jun 2019 HAL is a multi-disciplinary open access L’archive ouverte pluridisciplinaire HAL, est archive for the deposit and dissemination of sci- destinée au dépôt et à la diffusion de documents entific research documents, whether they are pub- scientifiques de niveau recherche, publiés ou non, lished or not. The documents may come from émanant des établissements d’enseignement et de teaching and research institutions in France or recherche français ou étrangers, des laboratoires abroad, or from public or private research centers. publics ou privés. Phobos, Deimos: Formation and Evolution Alex Soumbatov-Gur The moons are confirmed to be ejected parts of Mars’ crust. After explosive throwing out as cone-like rocks they plastically evolved with density decays and materials transformations. Their expansion evolutions were accompanied by global ruptures and small scale rock ejections with concurrent crater formations. The scenario reconciles orbital and physical parameters of the moons. It coherently explains dozens of their properties including spectra, appearances, size differences, crater locations, fracture symmetries, orbits, evolution trends, geologic activity, Phobos’ grooves, mechanism of their origin, etc. The ejective approach is also discussed in the context of observational data on near-Earth asteroids, main belt asteroids Steins, Vesta, and Mars. The approach incorporates known fission mechanism of formation of miniature asteroids, logically accounts for its outliers, and naturally explains formations of small celestial bodies of various sizes. -
Politikens Drivfjäder-Erik Bodensten
Politikens drivfjäder Frihetstidens partiberättelser och den moralpolitiska logiken BODENSTEN, ERIK 2016 Link to publication Citation for published version (APA): BODENSTEN, ERIK. (2016). Politikens drivfjäder: Frihetstidens partiberättelser och den moralpolitiska logiken. Lund University (Media-Tryck). Total number of authors: 1 General rights Unless other specific re-use rights are stated the following general rights apply: Copyright and moral rights for the publications made accessible in the public portal are retained by the authors and/or other copyright owners and it is a condition of accessing publications that users recognise and abide by the legal requirements associated with these rights. • Users may download and print one copy of any publication from the public portal for the purpose of private study or research. • You may not further distribute the material or use it for any profit-making activity or commercial gain • You may freely distribute the URL identifying the publication in the public portal Read more about Creative commons licenses: https://creativecommons.org/licenses/ Take down policy If you believe that this document breaches copyright please contact us providing details, and we will remove access to the work immediately and investigate your claim. LUND UNIVERSITY PO Box 117 221 00 Lund +46 46-222 00 00 STUDIA HISTORICA LUNDENSIA STUDIA HISTORICA LUNDENSIA Politikens drivfjäder FRIHETSTIDENS PARTIBERÄTTELSER OCH DEN MORALPOLITISKA LOGIKEN Erik Bodensten Hur kan den moraliskt fördärvade och lättkorrumperade människan upprätta ett stabilt självstyre? Vilka medborgare är tillräckligt dygdiga för att anförtros styret? Och hur kan de skiljas från de korrumperade? Dessa frågor var av central politisk betydelse för den förmoderna människan. De satte också sin prägel på det politiska livet under frihetstiden, då kungamakten var kraftigt försvagad och då antalet politiska deltagare mångfaldigades. -
Appendix I Lunar and Martian Nomenclature
APPENDIX I LUNAR AND MARTIAN NOMENCLATURE LUNAR AND MARTIAN NOMENCLATURE A large number of names of craters and other features on the Moon and Mars, were accepted by the IAU General Assemblies X (Moscow, 1958), XI (Berkeley, 1961), XII (Hamburg, 1964), XIV (Brighton, 1970), and XV (Sydney, 1973). The names were suggested by the appropriate IAU Commissions (16 and 17). In particular the Lunar names accepted at the XIVth and XVth General Assemblies were recommended by the 'Working Group on Lunar Nomenclature' under the Chairmanship of Dr D. H. Menzel. The Martian names were suggested by the 'Working Group on Martian Nomenclature' under the Chairmanship of Dr G. de Vaucouleurs. At the XVth General Assembly a new 'Working Group on Planetary System Nomenclature' was formed (Chairman: Dr P. M. Millman) comprising various Task Groups, one for each particular subject. For further references see: [AU Trans. X, 259-263, 1960; XIB, 236-238, 1962; Xlffi, 203-204, 1966; xnffi, 99-105, 1968; XIVB, 63, 129, 139, 1971; Space Sci. Rev. 12, 136-186, 1971. Because at the recent General Assemblies some small changes, or corrections, were made, the complete list of Lunar and Martian Topographic Features is published here. Table 1 Lunar Craters Abbe 58S,174E Balboa 19N,83W Abbot 6N,55E Baldet 54S, 151W Abel 34S,85E Balmer 20S,70E Abul Wafa 2N,ll7E Banachiewicz 5N,80E Adams 32S,69E Banting 26N,16E Aitken 17S,173E Barbier 248, 158E AI-Biruni 18N,93E Barnard 30S,86E Alden 24S, lllE Barringer 29S,151W Aldrin I.4N,22.1E Bartels 24N,90W Alekhin 68S,131W Becquerei -
Requirements and Limits for Life in the Context of Exoplanets
Requirements and limits for life in the context SPECIAL FEATURE of exoplanets Christopher P. McKay1 Space Science Division, National Aeronautics and Space Administration Ames Research Center, Moffett Field, CA 94035 Edited by Adam S. Burrows, Princeton University, Princeton, NJ, and accepted by the Editorial Board April 16, 2014 (received for review October 15, 2013) The requirements for life on Earth, its elemental composition, and bacteriorhodopsin, to convert photon energy to the energy of an its environmental limits provide a way to assess the habitability of electron which then completes a redox reaction. The electrons from exoplanets. Temperature is key both because of its influence on the redox reaction are used to create an electrochemical gradient liquid water and because it can be directly estimated from orbital across cell membranes (3). This occurs in the mitochondria in of and climate models of exoplanetary systems. Life can grow and most eukaryotes and in the cell membrane of prokaryotic cells. It reproduce at temperatures as low as −15 °C, and as high as 122 °C. has recently been shown that electrons provided directly as elec- Studies of life in extreme deserts show that on a dry world, even trical current can also drive microbial metabolism (4). Although life a small amount of rain, fog, snow, and even atmospheric humidity can detect and generate other energy sources including magnetic, can be adequate for photosynthetic production producing a small kinetic, gravitational, thermal gradient, and electrostatic, none of but detectable microbial community. Life is able to use light at levels these is used for metabolic energy. -
Eros' Rahe Dorsum
Meteoritics & Planetary Science 43, Nr 3, 435–449 (2008) AUTHOR’S PROOF Abstract available online at http://meteoritics.org Eros’ Rahe Dorsum: Implications for internal structure Richard GREENBERG Lunar and Planetary Laboratory, The University of Arizona, 1629 East University Blvd., Tucson, Arizona 85721, USA E-mail: [email protected] (Received 14 September 2006; revision accepted 14 August 2007) Abstract–An intriguing discovery of the NEAR imaging and laser-ranging experiments was the ridge system known as Rahe Dorsum and its possible relation with global-scale internal structure. The curved path of the ridge over the surface roughly defines a plane cutting through Eros. Another lineament on the other side of Eros, Calisto Fossae, seems to lie nearly on the same plane. The NEAR teams interpret Rahe as the expression of a compressive fault (a plane of weakness), because portions are a scarp, which on Earth would be indicative of horizontal compression, where shear displacement along a dipping fault has thrust the portion of the lithosphere on one side of the fault up relative to the other side. However, given the different geometry of Eros, a scarp may not have the same relationship to underlying structure as it does on Earth. The plane through Eros runs nearly parallel to, and just below, the surface facet adjacent to Rahe Dorsum. The plane then continues lengthwise through the elongated body, a surprising geometry for a plane of weakness on a battered body. Moreover, an assessment of the topography of Rahe Dorsum indicates that it is not consistent with displacement on the Rahe plane. -
Liquid Water on Mars
Report, Planetary Sciences Unit (AST80015), Swinburne Astronomy Online Preprint typeset using LATEX style emulateapj v. 12/16/11 LIQUID WATER ON MARS M. Usatov1 Report, Planetary Sciences Unit (AST80015), Swinburne Astronomy Online ABSTRACT Geomorphological, mineralogical and other evidence of the conditions favoring the existence of water on Mars in liquid phase is reviewed. This includes signatures of past and, possibly, present aqueous environments, such as the northern ocean, lacustrine environments, sedimentary and thermokarst landforms, glacial activity and water erosion features. Reviewed also are hydrous weathering processes, observed on surface remotely and also via analysis of Martian meteorites. Chemistry of Martian water is discussed: the triple point, salts and brines, as well as undercooled liquid interfacial and solid-state greenhouse effect melted waters that may still be present on Mars. Current understanding of the evolution of Martian hydrosphere over geological timescales is presented from early period to the present time, along with the discussion of alternative interpretations and possibilities of dry and wet Mars extremes. 1. INTRODUCTION morphological and mineralogical evidence of aqueous en- The presence of water on Earth, as seen from space, vironments available in the past and, possibly, present can be implied from the observations of low-albedo fea- time is presented in x3 which will be correlated with the tures, like seas and oceans, fluvial features on its sur- current understanding of the evolution of Martian hy- face, atmospheric phenomena, polar ice caps, and the drosphere at x4. Alternative (dry) interpretations of the snow cover exhibiting seasonal variations, not to mention evidence are discussed in x5. spectroscopy. -
Near Earth Asteroid Rendezvous: Mission Summary 351
Cheng: Near Earth Asteroid Rendezvous: Mission Summary 351 Near Earth Asteroid Rendezvous: Mission Summary Andrew F. Cheng The Johns Hopkins Applied Physics Laboratory On February 14, 2000, the Near Earth Asteroid Rendezvous spacecraft (NEAR Shoemaker) began the first orbital study of an asteroid, the near-Earth object 433 Eros. Almost a year later, on February 12, 2001, NEAR Shoemaker completed its mission by landing on the asteroid and acquiring data from its surface. NEAR Shoemaker’s intensive study has found an average density of 2.67 ± 0.03, almost uniform within the asteroid. Based upon solar fluorescence X-ray spectra obtained from orbit, the abundance of major rock-forming elements at Eros may be consistent with that of ordinary chondrite meteorites except for a depletion in S. Such a composition would be consistent with spatially resolved, visible and near-infrared (NIR) spectra of the surface. Gamma-ray spectra from the surface show Fe to be depleted from chondritic values, but not K. Eros is not a highly differentiated body, but some degree of partial melting or differentiation cannot be ruled out. No evidence has been found for compositional heterogeneity or an intrinsic magnetic field. The surface is covered by a regolith estimated at tens of meters thick, formed by successive impacts. Some areas have lesser surface age and were apparently more recently dis- turbed or covered by regolith. A small center of mass offset from the center of figure suggests regionally nonuniform regolith thickness or internal density variation. Blocks have a nonuniform distribution consistent with emplacement of ejecta from the youngest large crater. -
Final Report for a Robotic Exploration Mission to Mars and Phobos Argos
NASA-CR-197168 NASw-4435 /'/F/ 4 '_/e'7 t'/-q 1- Final Report for a Robotic Exploration Mission to Mars and Phobos PROJECT AENEAS Response to RFP Number ASE274L.0893 o r,4 u_ 4" submitted to: I ,-- ,0 U i'_ C_ e" 0 Z _ 0 Dr. George Botbyl The University of Texas at Austin Department of Aerospace Engineering and Engineering Mechanics Austin, Texas 78712 Z cn _. submitted by: 0 cO_ 0 Argos Space Endeavours 29 November 1993 zxI_f _rOos 8Face _.aea_ours _roJect Aeneas CDestBn _"eam Fall 1993 Chief Executive Officer Justin H. Kerr Chief Engineer Erin Defoss6 Chief Administrator Quang Ho Engineers Emisto Barriga Grant Davis Steve McCourt Matt Smith Aeneas Project Preliminary Design of a Robotic Exploration Mission to Mars and Phobos Approved: Justin H. Kerr CEO, Argos Space Endeavours Approved: Erin Defoss6 Chief Engineer, Argos Space Endeavours Approved: Quang Ho Administrative Officer, Argos Space Endeavours Argos 8pace q .aca ours University of Texas at Austin Department of Aerospace Engineering and Engineering Mechanics November 1993 Acknowledgments Argos Space Endeavours would like to thank all personnel at The University and in industry who made Project Aeneas possible. This project was conducted with the support of the NASA/USRA Advanced Design Program. Argos Space Endeavours wholeheartedly thanks the following faculty, staff, and students from the University of Texas at Austin: Dr. Wallace Fowler, Dr. Ronald Stearman, Dr. John Lundberg, Professor Richard Drury, Dr. David Dolling, Ms. Kelly Spears, Mr. Elfego Piton, Mr. Tony Economopoulos, and Mr. David Garza. The support of Project Aeneas from the aerospace industry was overwhelming. -
Asteroid Surface Geophysics
Asteroid Surface Geophysics Naomi Murdoch Institut Superieur´ de l’Aeronautique´ et de l’Espace (ISAE-SUPAERO) Paul Sanchez´ University of Colorado Boulder Stephen R. Schwartz University of Nice-Sophia Antipolis, Observatoire de la Coteˆ d’Azur Hideaki Miyamoto University of Tokyo The regolith-covered surfaces of asteroids preserve records of geophysical processes that have oc- curred both at their surfaces and sometimes also in their interiors. As a result of the unique micro-gravity environment that these bodies posses, a complex and varied geophysics has given birth to fascinating features that we are just now beginning to understand. The processes that formed such features were first hypothesised through detailed spacecraft observations and have been further studied using theoretical, numerical and experimental methods that often combine several scientific disciplines. These multiple approaches are now merging towards a further understanding of the geophysical states of the surfaces of asteroids. In this chapter we provide a concise summary of what the scientific community has learned so far about the surfaces of these small planetary bodies and the processes that have shaped them. We also discuss the state of the art in terms of experimental techniques and numerical simulations that are currently being used to investigate regolith processes occurring on small-body surfaces and that are contributing to the interpretation of observations and the design of future space missions. 1. INTRODUCTION wide range of geological features have been observed on the surfaces of asteroids and other small bodies such as the nu- Before the first spacecraft encounters with asteroids, cleus of comet 103P/Hartley 2 (Thomas et al. -
Phobos State of Knowledge and Rationales for Future Exploration
Phobos/Deimos State of Knowledge in Preparation for Future Exploration Julie Castillo-Rogez Laboratory for Frozen Astromaterials, PI NIAC for Hybrid Rovers/Hoppers, Study Scientist Jet Propulsion Laboratory, California Institute of Technology Initial Phobos/Deimos DRM Meeting for HAT Outline Introduction Science at Phobos Deimos Human Exploration Considerations Outline Introduction Science at Phobos Deimos Human Exploration Considerations Scope • Characterize Phobos/Deimos environment - Soil properties – mechanical and chemical - Surface dynamics - Subsurface properties (e.g., caves, regolith thickness) - Hazards – Radiations, topography, dust, electrostatic charging • Identify potential landing sites - Hazards – topography, surface dynamics - Vantage point wrt Mars - Scientific interest Moons Properties Phobos Deimos Shape (km) 26.8 × 22.4 × 18.4 15 × 12.2 × 10.4 Density (kg/m3) 1876 1471 Surface Gravity (Equator) µg 190-860 390 Escape Velocity (m/s) 11.3 5.6 SMA (km) 9,377 23,460 Eccentricity 0.015 1 0.000 2 Rotation Period (hr) 7h39.2 30h18 Forced Libration in Longitude 1.24±0.15 ?? (deg.) Orbital Period (hr) Synchronous Synchronous Equatorial Rotation Velocity 11.0 1.6 (km/h) – Longest axis Surface Temperature (K) 150-300 233 Outline Introduction Science at Phobos Deimos Human Exploration Considerations Key Science • Mysterious origin is endless subject of discussion – Remnant planetesimal vs. captured asteroid vs. Mars ejectas – Brings constraints on Mars’ early history and/or Solar system • Likely to have accumulated material from -
Exobiology in the Solar System & the Search for Life on Mars
SP-1231 SP-1231 October 1999 Exobiology in the Solar System & The Search for Life on Mars for The Search Exobiology in the Solar System & Exobiology in the Solar System & The Search for Life on Mars Report from the ESA Exobiology Team Study 1997-1998 Contact: ESA Publications Division c/o ESTEC, PO Box 299, 2200 AG Noordwijk, The Netherlands Tel. (31) 71 565 3400 - Fax (31) 71 565 5433 SP-1231 October 1999 EXOBIOLOGY IN THE SOLAR SYSTEM AND THE SEARCH FOR LIFE ON MARS Report from the ESA Exobiology Team Study 1997-1998 Cover Fossil coccoid bacteria, 1 µm in diameter, found in sediment 3.3-3.5 Gyr old from the Early Archean of South Africa. See pages 160-161. Background: a portion of the meandering canyons of the Nanedi Valles system viewed by Mars Global Surveyor. The valley is about 2.5 km wide; the scene covers 9.8 km by 27.9 km centred on 5.1°N/48.26°W. The valley floor at top right exhibits a 200 m-wide channel covered by dunes and debris. This channel suggests that the valley might have been carved by water flowing through the system over a long period, in a manner similar to rivers on Earth. (Malin Space Science Systems/NASA) SP-1231 ‘Exobiology in the Solar System and The Search for Life on Mars’, ISBN 92-9092-520-5 Scientific Coordinators: André Brack, Brian Fitton and François Raulin Edited by: Andrew Wilson ESA Publications Division Published by: ESA Publications Division ESTEC, Noordwijk, The Netherlands Price: 70 Dutch Guilders/ EUR32 Copyright: © 1999 European Space Agency Contents Foreword 7 I An Exobiological View of the -
A Sensitive Search for SO2 in the Martian Atmosphere: Implications for Seepage and Origin of Methane
Icarus 178 (2005) 487–492 www.elsevier.com/locate/icarus A sensitive search for SO2 in the martian atmosphere: Implications for seepage and origin of methane Vladimir A. Krasnopolsky ∗,1 Department of Physics, Catholic University of America, Washington, DC 20064, USA Received 2 December 2004; revised 29 April 2005 Available online 1 July 2005 Abstract −1 Mars was observed near the peak of the strongest SO2 band at 1364–1373 cm with resolving power of 77,000 using the Texas Echelon Cross Echelle Spectrograph on the NASA Infrared Telescope Facility. The observation covered the Tharsis volcano region which may be preferable to search for SO2. The spectrum shows absorption lines of three CO2 isotopomers and three H2O isotopomers. The water vapor abundance derived from the HDO lines assuming D/H = 5.5 times the terrestrial value is 12 ± 1.0 pr. µm, in agreement with the simultaneous ◦ ◦ MGS/TES observations of 14 pr. µm at the latitudes (50 Sto10 N) of our observation. Summing of spectral intervals at the expected positions of sixteen SO2 lines puts a 2σ upper limit on SO2 of 1 ppb. SO2 may be emitted into the martian atmosphere by seepage and is removed by three-body reactions with OH and O. The SO2 lifetime, 2 years, is longer than the global mixing time 0.5 year, so SO2 should be rather uniformly distributed across Mars. Seepage of SO2 is less than 15,000 tons per year on Mars which is smaller than the volcanic −4 −3 production of SO2 on the Earth by a factor of 700.