The Lifecycle of the Neuronal Microtubule Transport Machinery
Total Page:16
File Type:pdf, Size:1020Kb
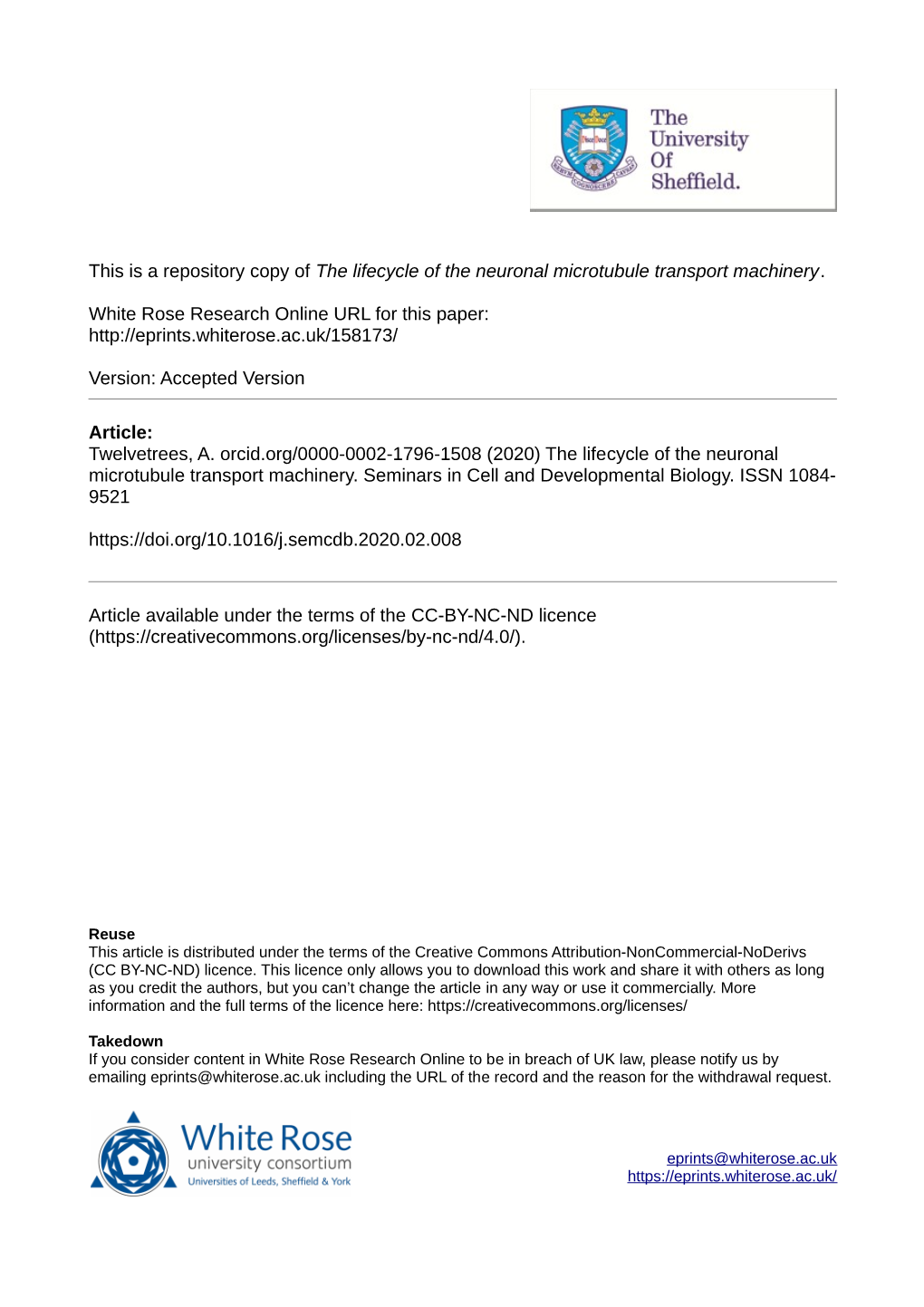
Load more
Recommended publications
-
Brain-Specific Expression of MAP2 Detected Using a Cloned Cdna Probe Sally A
View metadata, citation and similar papers at core.ac.uk brought to you by CORE provided by PubMed Central Brain-specific Expression of MAP2 Detected Using a Cloned cDNA Probe Sally A. Lewis,* Alfredo Villasante,* Peter Sherline,* and Nicholas J. Cowan* *Department of Biochemistry, New York University Medical Center, New York, New York 10016; *Division of Endocrinology, Cornell University Medical College, New York, New York 10021 Abstract. We describe the isolation of a set of over- consistent with the coding capacity required by a lapping cDNAs encoding mouse microtubule associ- 250,000-D protein. ated protein 2 (MAP2), using an anti-MAP antiserum The MAP2-specific cloned cDNA probes were used to screen a mouse brain cDNA expression library in RNA blot transfer experiments to assay for the cloned in bacteriophage hgt 11. The authenticity of presence of MAP2 mRNA in a variety of mouse tis- these clones was established by the following criteria: sues. Though brain contained abundant quantities of (a) three non-identical clones each expressing a MAP2 mRNA, no corresponding sequences were de- MAP2 immunoreactive fusion protein were independ- tectable in RNA prepared from liver, kidney, spleen, ently isolated from the expression library; each of stomach, or thymus. We conclude that the expression these clones cross-hybridized at the nucleic acid level; of MAP2 is brain-specific. (b) anti-MAP antiserum was affinity purified using Use of the MAP2 specific cDNA probes in genomic nitrocellulose-bound fusion protein; these antibodies Southern blot transfer experiments showed the pres- detected only MAP2 in an immunoblot experiment of ence of a single gene encoding MAP2 in mouse. -
A Computational Approach for Defining a Signature of Β-Cell Golgi Stress in Diabetes Mellitus
Page 1 of 781 Diabetes A Computational Approach for Defining a Signature of β-Cell Golgi Stress in Diabetes Mellitus Robert N. Bone1,6,7, Olufunmilola Oyebamiji2, Sayali Talware2, Sharmila Selvaraj2, Preethi Krishnan3,6, Farooq Syed1,6,7, Huanmei Wu2, Carmella Evans-Molina 1,3,4,5,6,7,8* Departments of 1Pediatrics, 3Medicine, 4Anatomy, Cell Biology & Physiology, 5Biochemistry & Molecular Biology, the 6Center for Diabetes & Metabolic Diseases, and the 7Herman B. Wells Center for Pediatric Research, Indiana University School of Medicine, Indianapolis, IN 46202; 2Department of BioHealth Informatics, Indiana University-Purdue University Indianapolis, Indianapolis, IN, 46202; 8Roudebush VA Medical Center, Indianapolis, IN 46202. *Corresponding Author(s): Carmella Evans-Molina, MD, PhD ([email protected]) Indiana University School of Medicine, 635 Barnhill Drive, MS 2031A, Indianapolis, IN 46202, Telephone: (317) 274-4145, Fax (317) 274-4107 Running Title: Golgi Stress Response in Diabetes Word Count: 4358 Number of Figures: 6 Keywords: Golgi apparatus stress, Islets, β cell, Type 1 diabetes, Type 2 diabetes 1 Diabetes Publish Ahead of Print, published online August 20, 2020 Diabetes Page 2 of 781 ABSTRACT The Golgi apparatus (GA) is an important site of insulin processing and granule maturation, but whether GA organelle dysfunction and GA stress are present in the diabetic β-cell has not been tested. We utilized an informatics-based approach to develop a transcriptional signature of β-cell GA stress using existing RNA sequencing and microarray datasets generated using human islets from donors with diabetes and islets where type 1(T1D) and type 2 diabetes (T2D) had been modeled ex vivo. To narrow our results to GA-specific genes, we applied a filter set of 1,030 genes accepted as GA associated. -
DYNC1I1 (NM 001135556) Human Tagged ORF Clone Product Data
OriGene Technologies, Inc. 9620 Medical Center Drive, Ste 200 Rockville, MD 20850, US Phone: +1-888-267-4436 [email protected] EU: [email protected] CN: [email protected] Product datasheet for RC226881 DYNC1I1 (NM_001135556) Human Tagged ORF Clone Product data: Product Type: Expression Plasmids Product Name: DYNC1I1 (NM_001135556) Human Tagged ORF Clone Tag: Myc-DDK Symbol: DYNC1I1 Synonyms: DNCI1; DNCIC1 Vector: pCMV6-Entry (PS100001) E. coli Selection: Kanamycin (25 ug/mL) Cell Selection: Neomycin This product is to be used for laboratory only. Not for diagnostic or therapeutic use. View online » ©2021 OriGene Technologies, Inc., 9620 Medical Center Drive, Ste 200, Rockville, MD 20850, US 1 / 4 DYNC1I1 (NM_001135556) Human Tagged ORF Clone – RC226881 ORF Nucleotide >RC226881 representing NM_001135556 Sequence: Red=Cloning site Blue=ORF Green=Tags(s) TTTTGTAATACGACTCACTATAGGGCGGCCGGGAATTCGTCGACTGGATCCGGTACCGAGGAGATCTGCC GCCGCGATCGCC ATGTCTGACAAAAGTGACTTAAAAGCTGAGCTAGAGCGCAAAAAGCAGCGCTTAGCACAGATAAGAGAAG AGAAGAAACGGAAGGAAGAGGAGAGGAAAAAGAAAGAGGCTGATATGCAGCAGAAGAAAGAACCCGTTCA GGACGACTCTGATCTGGATCGCAAACGACGAGAGACAGAGGCTTTGCTGCAAAGCATTGGTATCTCACCG GAGCCGCCTCTAGTCCCAACCCCTATGTCTCCCTCCTCGAAATCAGTGAGCACTCCCAGTGAAGCTGGAA GCCAAGACTCAGGCGATCTGGGGCCATTAACAAGGACCCTGCAGTGGGACACAGACCCCTCAGTGCTCCA GCTGCAGTCAGACTCAGAACTTGGAAGAAGACTGCATAAACTGGGCGTGTCAAAGGTCACCCAAGTGGAT TTCCTGCCAAGGGAAGTAGTGTCCTACTCAAAGGAGACCCAGACTCCTCTTGCCACGCATCAGTCTGAAG AGGATGAGGAAGATGAGGAAATGGTGGAATCTAAAGTTGGCCAGGACTCAGAACTGGAAAATCAGGACAA AAAACAGGAAGTGAAGGAAGCCCCTCCAAGAGAGTTGACAGAGGAAGAAAAACAGCAGATCATTCATTCA -
1 Metabolic Dysfunction Is Restricted to the Sciatic Nerve in Experimental
Page 1 of 255 Diabetes Metabolic dysfunction is restricted to the sciatic nerve in experimental diabetic neuropathy Oliver J. Freeman1,2, Richard D. Unwin2,3, Andrew W. Dowsey2,3, Paul Begley2,3, Sumia Ali1, Katherine A. Hollywood2,3, Nitin Rustogi2,3, Rasmus S. Petersen1, Warwick B. Dunn2,3†, Garth J.S. Cooper2,3,4,5* & Natalie J. Gardiner1* 1 Faculty of Life Sciences, University of Manchester, UK 2 Centre for Advanced Discovery and Experimental Therapeutics (CADET), Central Manchester University Hospitals NHS Foundation Trust, Manchester Academic Health Sciences Centre, Manchester, UK 3 Centre for Endocrinology and Diabetes, Institute of Human Development, Faculty of Medical and Human Sciences, University of Manchester, UK 4 School of Biological Sciences, University of Auckland, New Zealand 5 Department of Pharmacology, Medical Sciences Division, University of Oxford, UK † Present address: School of Biosciences, University of Birmingham, UK *Joint corresponding authors: Natalie J. Gardiner and Garth J.S. Cooper Email: [email protected]; [email protected] Address: University of Manchester, AV Hill Building, Oxford Road, Manchester, M13 9PT, United Kingdom Telephone: +44 161 275 5768; +44 161 701 0240 Word count: 4,490 Number of tables: 1, Number of figures: 6 Running title: Metabolic dysfunction in diabetic neuropathy 1 Diabetes Publish Ahead of Print, published online October 15, 2015 Diabetes Page 2 of 255 Abstract High glucose levels in the peripheral nervous system (PNS) have been implicated in the pathogenesis of diabetic neuropathy (DN). However our understanding of the molecular mechanisms which cause the marked distal pathology is incomplete. Here we performed a comprehensive, system-wide analysis of the PNS of a rodent model of DN. -
Structure of the Molecular Chaperone Prefoldin
CORE Metadata, citation and similar papers at core.ac.uk Provided by Elsevier - Publisher Connector Cell, Vol. 103, 621±632, November 10, 2000, Copyright 2000 by Cell Press Structure of the Molecular Chaperone Prefoldin: Unique Interaction of Multiple Coiled Coil Tentacles with Unfolded Proteins of newly synthesized bacterial %10ف ,Ralf Siegert,² Michel R. Leroux,²³ 1999). In addition Clemens Scheufler, F. Ulrich Hartl,* proteins complete their folding in the sequestered envi- and Ismail Moarefi* ronment provided by GroEL/GroES (Horwich et al., 1993; Max-Planck Institut fuÈ r Biochemie Ewalt et al., 1997; Houry et al., 1999). The eukaryotic Am Klopferspitz 18a Hsp70 chaperone machine also binds nascent chains D82152 Martinsried (Beckmann et al., 1990; Nelson et al., 1992; Eggers et Germany al., 1997; Thulasiraman et al., 1999), and some proteins, including actins and tubulins, depend on the Group II cytosolic chaperonin TRiC (TCP-1 ring Complex; also Summary termed CCT) for folding (Frydman et al., 1992; Gao et al., 1992; Yaffe et al., 1992; Kubota et al., 1995; Siegers Prefoldin (GimC) is a hexameric molecular chaperone et al., 1999). complex built from two related classes of subunits The archaeal Group II chaperonin (thermosome) is and present in all eukaryotes and archaea. Prefoldin closely related to its eukaryotic homologue TRiC (Gutsche et al., 1999). In contrast, Hsp70 proteins and interacts with nascent polypeptide chains and, in vitro, TF are generally missing from the archaeal kingdom can functionally substitute for the Hsp70 chaperone though some archaea have acquired Hsp70, presumably system in stabilizing non-native proteins for subse- by lateral gene transfer (Gribaldo et al., 1999). -
Targeting Tyro3 Ameliorates a Model of PGRN-Mutant FTLD-TDP Via Tau
ARTICLE DOI: 10.1038/s41467-018-02821-z OPEN Targeting Tyro3 ameliorates a model of PGRN- mutant FTLD-TDP via tau-mediated synaptic pathology Kyota Fujita1, Xigui Chen1, Hidenori Homma1, Kazuhiko Tagawa1, Mutsuki Amano 2, Ayumu Saito3, Seiya Imoto 4, Hiroyasu Akatsu5, Yoshio Hashizume6, Kozo Kaibuchi2, Satoru Miyano3 & Hitoshi Okazawa1 PGRN 1234567890():,; Mutations in the progranulin ( ) gene cause a tau pathology-negative and TDP43 pathology-positive form of frontotemporal lobar degeneration (FTLD-TDP). We generated a knock-in mouse harboring the R504X mutation (PGRN-KI). Phosphoproteomic analysis of this model revealed activation of signaling pathways connecting PKC and MAPK to tau prior to TDP43 aggregation and cognitive impairments, and identified PKCα as the kinase responsible for the early-stage tau phosphorylation at Ser203. Disinhibition of Gas6 binding to Tyro3 due to PGRN reduction results in activation of PKCα via PLCγ, inducing tau phosphorylation at Ser203, mislocalization of tau to dendritic spines, and spine loss. Administration of a PKC inhibitor, B-Raf inhibitor, or knockdown of molecules in the Gas6-Tyro3-tau axis rescues spine loss and cognitive impairment of PGRN-KI mice. Collectively, these results suggest that targeting of early-stage and aggregation-independent tau signaling represents a promising therapeutic strategy for this disease. 1 Department of Neuropathology, Medical Research Institute and Center for Brain Integration Research, Tokyo Medical and Dental University, 1-5-45 Yushima, Bunkyo-ku, Tokyo 113-8510, Japan. 2 Department of Cell Pharmacology, Graduate School of Medicine, Nagoya University, 65, Tsurumai, Showa, Nagoya, Aichi 466-8550, Japan. 3 Human Genome Center, Institute of Medical Science, The University of Tokyo, 4-6-1, Shirokanedai, Minato-ku, Tokyo 108- 8639, Japan. -
Immunolocalization and Molecular Properties of a High Molecular Weight Microtubule-Bundling Protein (Syncolin) from Chicken Erythrocytes E Feick, R
Immunolocalization and Molecular Properties of a High Molecular Weight Microtubule-bundling Protein (Syncolin) from Chicken Erythrocytes E Feick, R. Foisner, and G. Wiche Institute of Biochemistry, University of Vienna, 1090 Vienna, Austria Abstract. A protein of apparent molecular weight was displaced from the polymer by salt. Brain as well 280,000 (syncolin), which is immunoreactive with an- as erythrocyte microtubules, reconstituted with taxol tibodies to hog brain microtubule-associated protein from MAP-free tubulin and purified syncolin, were ag- (MAP) 2, was purified from chicken erythrocytes. Im- gregated into dense bundles containing up to 15 Downloaded from http://rupress.org/jcb/article-pdf/112/4/689/1060909/689.pdf by guest on 29 September 2021 munofluorescence microscopy of bone marrow cells microtubules, as determinedby'etectron microscopy. revealed the presence of syncolin in cells at all stages On the ultrastructural level, syncolin molecules were of erythrocyte differentiation. In early erythroblasts visualized as globular or ringlike structures, in con- syncolin was diffusely distributed throughout the trast to the thin, threadlike appearance of filamentous cytoplasm. At later stages it was found along microtu- MAPs, such as brain MAP 2. According to ultrastruc- bules of the marginal band, as confirmed by immuno- tural measurements and gel permeation chromatogra- electron microscopy. The association of syncolin with phy, syncolin's molecular weight was ,',,1 x 106. It is the marginal band was dependent on the integrity of suggested that syncolin's specific function is the cross- microtubules, as demonstrated by temperature- linking of microtubules in the marginal band and, by dependent de- and repolymerization or marginal band implication, the stabilization of this structure typical microtubules. -
And High-Molecular-Weight Forms of MAP2 in the Developing Cerebellum
The Journal of Neuroscience, December 1999, 8(12): 4503-4512 The Sequential Appearance of Low- and High-Molecular-Weight Forms of MAP2 in the Developing Cerebellum R. P. Tucker,’ L. I. Binder,’ C. Viereck,’ B. A. Hemmings,’ and A. I. Matus’ ‘Friedrich Miescher Institute, CH-4002 Basel, Switzerland, and *Department of Cell Biology and Anatomy, School of Medicine and Dentistry, University of Alabama at Birmingham, Birmingham, Alabama 35294 Mammalian microtubule-associated protein 2 (MAPS) exists shown that a number of MAPS promote the assemblyof tubulin in high-molecular-weight (M, -280,000) and low-molecular- into microtubulesand stabilize the resulting microtubulesagainst weight (M, -70,000) forms, with the latter protein being more the depolymerizing effects of cold and various pharmacological abundant in embryonic brain homogenates than in prepa- agents(Olmsted, 1986; Matus, 1988). The most abundant MAP rations from mature brain (Riederer and Matus, 1985). In the in the mammalian CNS, MAP2, displays each of these char- current study, we have shown that avian MAP2 also exists acteristics: (1) There are 2 closely related high-molecular-weight as both high- (M, -260,000) and low-molecular-weight (M, (HMW) forms of MAP2, MAP2a, and MAP2b, which change -65,000) forms whose relative abundance changes during in relative abundanceduring development (Binder et al., 1984; brain maturation, indicating a conserved function for these Burgoyne and Cummings, 1984);(2) MAP2 is found in dendrites proteins during vertebrate neuronal morphogenesis. Using and only rarely in axons (e.g., Cacereset al., 1984; DeCamilli indirect immunohistochemistry, we have determined the cel- et al., 1984; Huber and Matus, 1984b); and (3) MAP2 stabilizes lular distribution of the high- and low-molecular-weight forms and promotes the assembly of microtubules in vitro (Murphy of MAP2 in the developing avian cerebellum. -
Investigation of Candidate Genes and Mechanisms Underlying Obesity
Prashanth et al. BMC Endocrine Disorders (2021) 21:80 https://doi.org/10.1186/s12902-021-00718-5 RESEARCH ARTICLE Open Access Investigation of candidate genes and mechanisms underlying obesity associated type 2 diabetes mellitus using bioinformatics analysis and screening of small drug molecules G. Prashanth1 , Basavaraj Vastrad2 , Anandkumar Tengli3 , Chanabasayya Vastrad4* and Iranna Kotturshetti5 Abstract Background: Obesity associated type 2 diabetes mellitus is a metabolic disorder ; however, the etiology of obesity associated type 2 diabetes mellitus remains largely unknown. There is an urgent need to further broaden the understanding of the molecular mechanism associated in obesity associated type 2 diabetes mellitus. Methods: To screen the differentially expressed genes (DEGs) that might play essential roles in obesity associated type 2 diabetes mellitus, the publicly available expression profiling by high throughput sequencing data (GSE143319) was downloaded and screened for DEGs. Then, Gene Ontology (GO) and REACTOME pathway enrichment analysis were performed. The protein - protein interaction network, miRNA - target genes regulatory network and TF-target gene regulatory network were constructed and analyzed for identification of hub and target genes. The hub genes were validated by receiver operating characteristic (ROC) curve analysis and RT- PCR analysis. Finally, a molecular docking study was performed on over expressed proteins to predict the target small drug molecules. Results: A total of 820 DEGs were identified between -
Modulating Hallmarks of Cholangiocarcinoma
University of Nebraska Medical Center DigitalCommons@UNMC Theses & Dissertations Graduate Studies Fall 12-14-2018 Modulating Hallmarks of Cholangiocarcinoma Cody Wehrkamp University of Nebraska Medical Center Follow this and additional works at: https://digitalcommons.unmc.edu/etd Part of the Molecular Biology Commons Recommended Citation Wehrkamp, Cody, "Modulating Hallmarks of Cholangiocarcinoma" (2018). Theses & Dissertations. 337. https://digitalcommons.unmc.edu/etd/337 This Dissertation is brought to you for free and open access by the Graduate Studies at DigitalCommons@UNMC. It has been accepted for inclusion in Theses & Dissertations by an authorized administrator of DigitalCommons@UNMC. For more information, please contact [email protected]. MODULATING HALLMARKS OF CHOLANGIOCARCINOMA by Cody J. Wehrkamp A DISSERTATION Presented to the Faculty of the University of Nebraska Graduate College in Partial Fulfillment of the Requirements for the Degree of Doctor of Philosophy Biochemistry and Molecular Biology Graduate Program Under the Supervision of Professor Justin L. Mott University of Nebraska Medical Center Omaha, Nebraska November 2018 Supervisory Committee: Kaustubh Datta, Ph.D. Melissa Teoh‐Fitzgerald, Ph.D. Richard G. MacDonald, Ph.D. Acknowledgements This endeavor has led to scientific as well as personal growth for me. I am indebted to many for their knowledge, influence, and support along the way. To my mentor, Dr. Justin L. Mott, you have been an incomparable teacher and invaluable guide. You upheld for me the concept that science is intrepid, even when the experience is trying. Through my training, and now here at the end, I can say that it has been an honor to be your protégé. When you have shaped your future graduates to be and do great, I will be privileged to say that I was your first one. -
Genetic and Genomic Analysis of Hyperlipidemia, Obesity and Diabetes Using (C57BL/6J × TALLYHO/Jngj) F2 Mice
University of Tennessee, Knoxville TRACE: Tennessee Research and Creative Exchange Nutrition Publications and Other Works Nutrition 12-19-2010 Genetic and genomic analysis of hyperlipidemia, obesity and diabetes using (C57BL/6J × TALLYHO/JngJ) F2 mice Taryn P. Stewart Marshall University Hyoung Y. Kim University of Tennessee - Knoxville, [email protected] Arnold M. Saxton University of Tennessee - Knoxville, [email protected] Jung H. Kim Marshall University Follow this and additional works at: https://trace.tennessee.edu/utk_nutrpubs Part of the Animal Sciences Commons, and the Nutrition Commons Recommended Citation BMC Genomics 2010, 11:713 doi:10.1186/1471-2164-11-713 This Article is brought to you for free and open access by the Nutrition at TRACE: Tennessee Research and Creative Exchange. It has been accepted for inclusion in Nutrition Publications and Other Works by an authorized administrator of TRACE: Tennessee Research and Creative Exchange. For more information, please contact [email protected]. Stewart et al. BMC Genomics 2010, 11:713 http://www.biomedcentral.com/1471-2164/11/713 RESEARCH ARTICLE Open Access Genetic and genomic analysis of hyperlipidemia, obesity and diabetes using (C57BL/6J × TALLYHO/JngJ) F2 mice Taryn P Stewart1, Hyoung Yon Kim2, Arnold M Saxton3, Jung Han Kim1* Abstract Background: Type 2 diabetes (T2D) is the most common form of diabetes in humans and is closely associated with dyslipidemia and obesity that magnifies the mortality and morbidity related to T2D. The genetic contribution to human T2D and related metabolic disorders is evident, and mostly follows polygenic inheritance. The TALLYHO/ JngJ (TH) mice are a polygenic model for T2D characterized by obesity, hyperinsulinemia, impaired glucose uptake and tolerance, hyperlipidemia, and hyperglycemia. -
Serum Albumin OS=Homo Sapiens
Protein Name Cluster of Glial fibrillary acidic protein OS=Homo sapiens GN=GFAP PE=1 SV=1 (P14136) Serum albumin OS=Homo sapiens GN=ALB PE=1 SV=2 Cluster of Isoform 3 of Plectin OS=Homo sapiens GN=PLEC (Q15149-3) Cluster of Hemoglobin subunit beta OS=Homo sapiens GN=HBB PE=1 SV=2 (P68871) Vimentin OS=Homo sapiens GN=VIM PE=1 SV=4 Cluster of Tubulin beta-3 chain OS=Homo sapiens GN=TUBB3 PE=1 SV=2 (Q13509) Cluster of Actin, cytoplasmic 1 OS=Homo sapiens GN=ACTB PE=1 SV=1 (P60709) Cluster of Tubulin alpha-1B chain OS=Homo sapiens GN=TUBA1B PE=1 SV=1 (P68363) Cluster of Isoform 2 of Spectrin alpha chain, non-erythrocytic 1 OS=Homo sapiens GN=SPTAN1 (Q13813-2) Hemoglobin subunit alpha OS=Homo sapiens GN=HBA1 PE=1 SV=2 Cluster of Spectrin beta chain, non-erythrocytic 1 OS=Homo sapiens GN=SPTBN1 PE=1 SV=2 (Q01082) Cluster of Pyruvate kinase isozymes M1/M2 OS=Homo sapiens GN=PKM PE=1 SV=4 (P14618) Glyceraldehyde-3-phosphate dehydrogenase OS=Homo sapiens GN=GAPDH PE=1 SV=3 Clathrin heavy chain 1 OS=Homo sapiens GN=CLTC PE=1 SV=5 Filamin-A OS=Homo sapiens GN=FLNA PE=1 SV=4 Cytoplasmic dynein 1 heavy chain 1 OS=Homo sapiens GN=DYNC1H1 PE=1 SV=5 Cluster of ATPase, Na+/K+ transporting, alpha 2 (+) polypeptide OS=Homo sapiens GN=ATP1A2 PE=3 SV=1 (B1AKY9) Fibrinogen beta chain OS=Homo sapiens GN=FGB PE=1 SV=2 Fibrinogen alpha chain OS=Homo sapiens GN=FGA PE=1 SV=2 Dihydropyrimidinase-related protein 2 OS=Homo sapiens GN=DPYSL2 PE=1 SV=1 Cluster of Alpha-actinin-1 OS=Homo sapiens GN=ACTN1 PE=1 SV=2 (P12814) 60 kDa heat shock protein, mitochondrial OS=Homo