A Conserved Stem of the Myxococcus Xanthus Srna Pxr Controls Srna
Total Page:16
File Type:pdf, Size:1020Kb
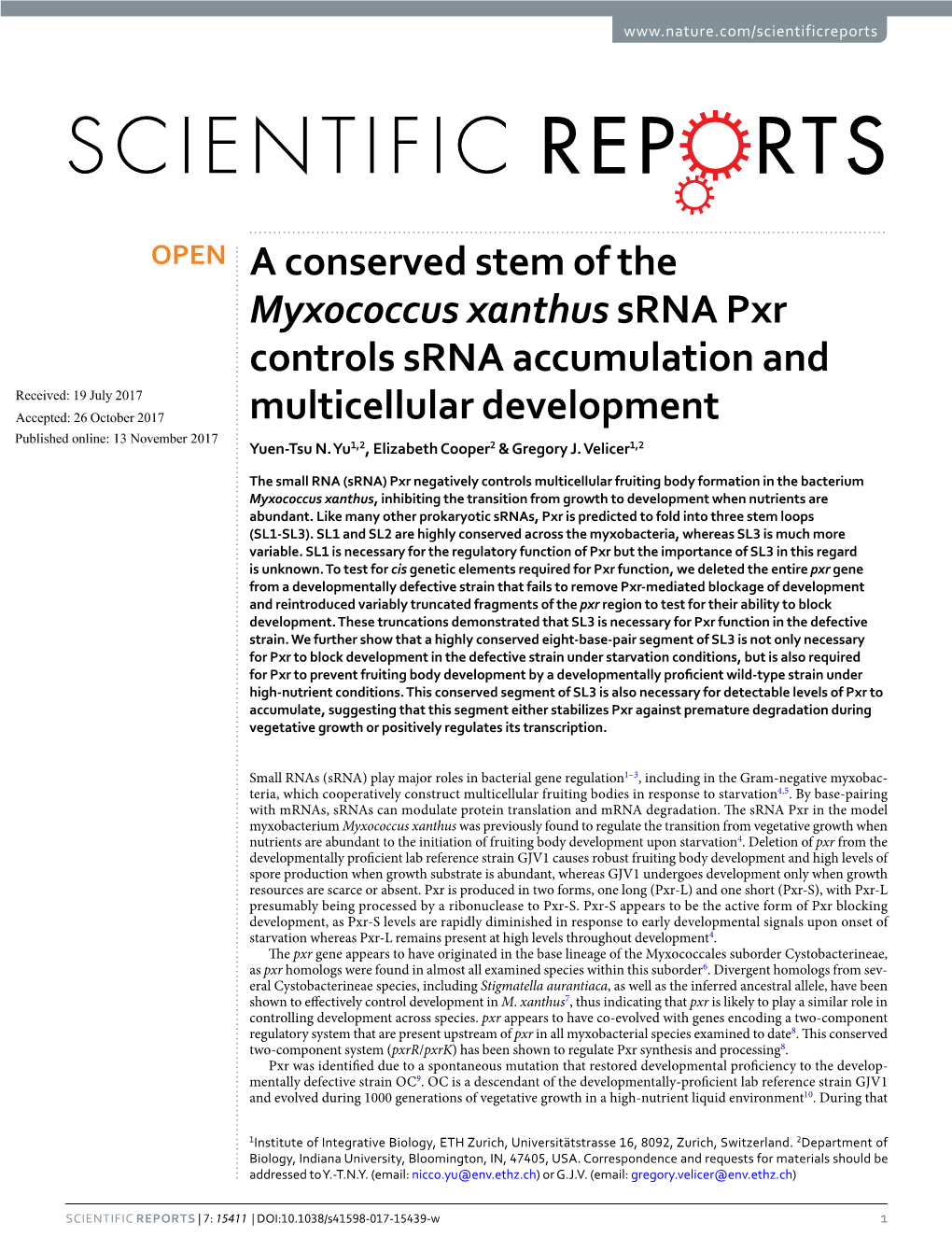
Load more
Recommended publications
-
Genomic Signatures of Predatory Bacteria
The ISME Journal (2013) 7, 756–769 & 2013 International Society for Microbial Ecology All rights reserved 1751-7362/13 www.nature.com/ismej ORIGINAL ARTICLE By their genes ye shall know them: genomic signatures of predatory bacteria Zohar Pasternak1, Shmuel Pietrokovski2, Or Rotem1, Uri Gophna3, Mor N Lurie-Weinberger3 and Edouard Jurkevitch1 1Department of Plant Pathology and Microbiology, The Hebrew University of Jerusalem, Rehovot, Israel; 2Department of Molecular Genetics, Weizmann Institute of Science, Rehovot, Israel and 3Department of Molecular Microbiology and Biotechnology, George S. Wise Faculty of Life Sciences, Tel Aviv University, Tel Aviv, Israel Predatory bacteria are taxonomically disparate, exhibit diverse predatory strategies and are widely distributed in varied environments. To date, their predatory phenotypes cannot be discerned in genome sequence data thereby limiting our understanding of bacterial predation, and of its impact in nature. Here, we define the ‘predatome,’ that is, sets of protein families that reflect the phenotypes of predatory bacteria. The proteomes of all sequenced 11 predatory bacteria, including two de novo sequenced genomes, and 19 non-predatory bacteria from across the phylogenetic and ecological landscapes were compared. Protein families discriminating between the two groups were identified and quantified, demonstrating that differences in the proteomes of predatory and non-predatory bacteria are large and significant. This analysis allows predictions to be made, as we show by confirming from genome data an over-looked bacterial predator. The predatome exhibits deficiencies in riboflavin and amino acids biosynthesis, suggesting that predators obtain them from their prey. In contrast, these genomes are highly enriched in adhesins, proteases and particular metabolic proteins, used for binding to, processing and consuming prey, respectively. -
Evolution and Design Governing Signal Precision and Amplification
Evolution and Design Governing Signal Precision and Amplification in a Bacterial Chemosensory Pathway Mathilde Guzzo, Rym Agrebi, Leon Espinosa, Gregory Baronian, Virginie Molle, Emilia M. F. Mauriello, Céline Brochier-Armanet, Tam Mignot To cite this version: Mathilde Guzzo, Rym Agrebi, Leon Espinosa, Gregory Baronian, Virginie Molle, et al.. Evolution and Design Governing Signal Precision and Amplification in a Bacterial Chemosensory Pathway. PLoS Genetics, Public Library of Science, 2015, 11 (8), pp.e1005460. 10.1371/journal.pgen.1005460. hal- 01452074 HAL Id: hal-01452074 https://hal.archives-ouvertes.fr/hal-01452074 Submitted on 27 Sep 2018 HAL is a multi-disciplinary open access L’archive ouverte pluridisciplinaire HAL, est archive for the deposit and dissemination of sci- destinée au dépôt et à la diffusion de documents entific research documents, whether they are pub- scientifiques de niveau recherche, publiés ou non, lished or not. The documents may come from émanant des établissements d’enseignement et de teaching and research institutions in France or recherche français ou étrangers, des laboratoires abroad, or from public or private research centers. publics ou privés. Distributed under a Creative Commons Attribution| 4.0 International License RESEARCH ARTICLE Evolution and Design Governing Signal Precision and Amplification in a Bacterial Chemosensory Pathway Mathilde Guzzo1☯, Rym Agrebi1☯¤, Leon Espinosa1, Grégory Baronian2, Virginie Molle2, Emilia M. F. Mauriello1, Céline Brochier-Armanet3, Tâm Mignot1* 1 Laboratoire de Chimie Bactérienne, Institut de Microbiologie de la Méditerranée, CNRS Aix-Marseille University UMR 7283, Marseille, France, 2 Laboratoire de Dynamique des Interactions Membranaires Normales et Pathologiques, CNRS Universités de Montpellier II et I, UMR 5235, case 107, Montpellier, France, 3 Université de Lyon, Université Lyon 1, CNRS, UMR5558, Laboratoire de Biométrie et Biologie Evolutive, Villeurbanne, France ☯ These authors contributed equally to this work. -
Novel Myxobacteria As a Potential Source of Natural Products and Description of Inter-Species Nature of C-Signal
Novel myxobacteria as a potential source of natural products and description of inter-species nature of C-signal Dissertation zur Erlangung des Grades des Doktors der Naturwissenschaften der Naturwissenschaftlich-Technischen Fakultät III Chemie, Pharmazie, Bio- und Werkstoffwissenschaften der Universität des Saarlandes von Ram Prasad Awal (M. Sc. in Medical Microbiology) Saarbrücken 2016 Tag des Kolloquiums: ......19.12.2016....................................... Dekan: ......Prof. Dr. Guido Kickelbick.............. Berichterstatter: ......Prof. Dr. Rolf Müller...................... ......Prof. Dr. Manfred J. Schmitt........... ............................................................... Vositz: ......Prof. Dr. Uli Kazmaier..................... Akad. Mitarbeiter: ......Dr. Jessica Stolzenberger.................. iii Diese Arbeit entstand unter der Anleitung von Prof. Dr. Rolf Müller in der Fachrichtung 8.2, Pharmazeutische Biotechnologie der Naturwissenschaftlich-Technischen Fakultät III der Universität des Saarlandes von Oktober 2012 bis September 2016. iv Acknowledgement Above all, I would like to express my special appreciation and thanks to my advisor Professor Dr. Rolf Müller. It has been an honor to be his Ph.D. student and work in his esteemed laboratory. I appreciate for his supervision, inspiration and for allowing me to grow as a research scientist. Your guidance on both research as well as on my career have been invaluable. I would also like to thank Professor Dr. Manfred J. Schmitt for his scientific support and suggestions to my research work. I am thankful for my funding sources that made my Ph.D. work possible. I was funded by Deutscher Akademischer Austauschdienst (DAAD) for 3 and half years and later on by Helmholtz-Institute. Many thanks to my co-advisors: Dr. Carsten Volz, who supported and guided me through the challenging research and Dr. Ronald Garcia for introducing me to the wonderful world of myxobacteria. -
Polyamine Distribution Profiles Among Some Members Within Delta-And Epsilon-Subclasses of Proteobacteria
Microbiol. Cult. Coll. June. 2004. p. 3 ― 8 Vol. 20, No. 1 Polyamine Distribution Profiles among Some Members within Delta-and Epsilon-Subclasses of Proteobacteria Koei Hamana1)*, Tomoko Saito1), Mami Okada1), and Masaru Niitsu2) 1)Department of Laboratory Sciences, School of Health Sciences, Faculty of Medicine, Gunma University, 39- 15 Showa-machi 3-chome, Maebashi, Gunma 371-8514, Japan 2)Faculty of Pharmaceutical Sciences, Josai University, Keyakidai 1-chome-1, Sakado, Saitama 350-0295, Japan Cellular polyamines of 18 species(13 genera)belonging to the delta and epsilon subclasses of the class Proteobacteria were analyzed by HPLC and GC. In the delta subclass, the four marine myxobacteria(the order Myxococcales), Enhygromyxa salina, Haliangium ochroceum, Haliangium tepidum and Plesiocystis pacifica contained spermidine. Fe(III)-reducing two Geobacter species and two Pelobacter species belonging to the order Desulfuromonadales con- tained spermidine. Bdellovibrio bacteriovorus was absent in cellular polyamines. Bacteriovorax starrii contained putrescine and spermidine. Bacteriovorax stolpii contained spermidine and homo- spermidine. Spermidine was the major polyamine in the sulfate-reducing delta proteobacteria belonging to the genera Desulfovibrio, Desulfacinum, Desulfobulbus, Desulfococcus and Desulfurella, and some species of them contained cadaverine. Within the epsilon subclass, three Sulfurospirillum species ubiquitously contained spermidine and one of the three contained sper- midine and cadaverine. Thiomicrospora denitrificans contained cadaverine and spermidine as the major polyamine. These data show that cellular polyamine profiles can be used as a chemotaxonomic marker within delta and epsilon subclasses. Key words: polyamine, spermidine, homospermidine, Proteobacteria The class Proteobacteria is a major taxon of the 18, 26). Fe(Ⅲ)-reducing members belonging to the gen- domain Bacteria and is phylogenetically divided into the era Pelobacter, Geobacter, Desulfuromonas and alpha, beta, gamma, delta and epsilon subclasses. -
The Correlation Between Morphological and Phylogenetic Classification of Myxobacteria
International Journal of Systematic Bacteriology (1 999), 49, 1255-1 262 Printed in Great Britain The correlation between morphological and phylogenetic classification of myxobacteria Cathrin Sproer,’ Hans Reichenbach’ and Erko Stackebrandtl Author for correspondence: Erko Stackebrandt.Tel: +49 531 2616 352. Fax: +49 531 2616 418. e-mail : [email protected] DSMZ-Deutsche Sammlung In order to determine whether morphological criteria are suitable to affiliate von Mikroorganismen und myxobacterial strains to species, a phylogenetic analysis of 16s rDNAs was Zellkulturen GmbH1 and G BF-Gesel Isc haft fur performed on 54 myxobacterial strains that represented morphologically 21 Biotechnologische species of the genera Angiococcus, Archangium, Chondromyces, Cystobacter, Forschung mbH*, 381 24 Melittangium, Myxococcus, Polyangium and Stigmatella, five invalid species Braunschweig, Germany and three unclassified isolates. The analysis included 12 previously published sequences. The branching pattern confirmed the deep trifurcation of the order Myxococcales. One lineage is defined by the genera Cystobacter, Angiococcus, Archangium, Melittangium, Myxococcus and Stigmatella. The study confirms the genus status of Corallococcus’, previously ‘Chondrococcus’,within the family Myxococcaceae. The second lineage contains the genus Chondromyces and the species Polyangium (‘Sorangium’) cellulosum, while the third lineage is comprised of Nannocystis and a strain identified as Polyangium vitellinum. With the exception of a small number of strains that did not cluster phylogenetically with members of the genus to which they were assigned by morphological criteria (‘Polyangium thaxteri’ PI t3, Polyangium cellulosum ATCC 25531T, Melittangium lichenicola ATCC 25947Tand Angiococcus disciformis An dl), the phenotypic classification should provide a sound basis for the description of neotype species in those cases where original strain material is not available or is listed as reference material. -
Niranjan Parajuli Et Al Paper
Journal of Institute of Science and Technology, 24(2), 7-16 (2019) ISSN: 2469-9062 (print), 2467-9240 (e) © IOST, Tribhuvan University Doi: http://doi.org/10.3126/jist.v24i2.27246 Research Article ISOLATION AND CHARACTERIZATION OF SOIL MYXOBACTERIA FROM NEPAL Nabin Rana1, Saraswoti Khadka1, Bishnu Prasad Marasini1, Bishnu Joshi1, Pramod Poudel2, Santosh Khanal1, Niranjan Parajuli3 1Department of Biotechnology, National College, Tribhuvan University, Naya Bazar, Kathmandu, Nepal 2Research Division, University Grant Commission (UGC-Nepal), Sanothimi, Bhaktapur, Nepal 3Central Department of Chemistry, Tribhuvan University, Kirtipur, Kathmandu, Nepal *Corresponding author: [email protected] (Received: May 22, 2019; Revised: October 26, 2019; Accepted: November 1, 2019) ABSTRACT Realizing myxobacteria as a potential source of antimicrobial metabolites, we pursued research to isolate myxobacteria showing antimicrobial properties. We have successfully isolated three strains (NR-1, NR-2, NR-3) using the Escherichia coli baiting technique. These isolates showed typical myxobacterial growth characteristics. Phylogenetic analysis showed that all the strains (NR-1, NR-2, NR-3) belong to the family Archangiaceae, suborder Cystobacterineae, and order Myxococcales. Furthermore, 16S rRNA gene sequence similarity searched through BLAST revealed that strain NR-1 showed the closest similarity (91.8 %) to the type strain Vitiosangium cumulatum (NR-156939), NR-2 showed (98.8 %) to the type of Cystobacter badius (NR-043940), and NR-3 showed the closest similarity (83.5 %) to the type of strain Cystobacter fuscus (KP-306730). All isolates showed better growth in 0.5-1 % NaCl and pH around 7.0, whereas no growth was observed at pH 9.0 and below 5.0. All strains showed better growth at 32° C and hydrolyzed starch, whereas casein was efficiently hydrolyzed by NR-1 and NR-2. -
Phylogenetic Profile of Copper Homeostasis in Deltaproteobacteria
Phylogenetic Profile of Copper Homeostasis in Deltaproteobacteria A Major Qualifying Report Submitted to the Faculty of Worcester Polytechnic Institute In Partial Fulfillment of the Requirements for the Degree of Bachelor of Science By: __________________________ Courtney McCann Date Approved: _______________________ Professor José M. Argüello Biochemistry WPI Project Advisor 1 Abstract Copper homeostasis is achieved in bacteria through a combination of copper chaperones and transporting and chelating proteins. Bioinformatic analyses were used to identify which of these proteins are present in Deltaproteobacteria. The genetic environment of the bacteria is affected by its lifestyle, as those that live in higher concentrations of copper have more of these proteins. Two major transport proteins, CopA and CusC, were found to cluster together frequently in the genomes and appear integral to copper homeostasis in Deltaproteobacteria. 2 Acknowledgements I would like to thank Professor José Argüello for giving me the opportunity to work in his lab and do some incredible research with some equally incredible scientists. I need to give all of my thanks to my supervisor, Dr. Teresita Padilla-Benavides, for having me as her student and teaching me not only lab techniques, but also how to be scientist. I would also like to thank Dr. Georgina Hernández-Montes and Dr. Brenda Valderrama from the Insituto de Biotecnología at Universidad Nacional Autónoma de México (IBT-UNAM), Campus Morelos for hosting me and giving me the opportunity to work in their lab. I would like to thank Sarju Patel, Evren Kocabas, and Jessica Collins, whom I’ve worked alongside in the lab. I owe so much to these people, and their support and guidance has and will be invaluable to me as I move forward in my education and career. -
A Recent Evolutionary Origin of a Bacterial Small RNA That Controls
Molecular Phylogenetics and Evolution 73 (2014) 1–9 Contents lists available at ScienceDirect Molecular Phylogenetics and Evolution journal homepage: www.elsevier.com/locate/ympev A recent evolutionary origin of a bacterial small RNA that controls multicellular fruiting body development q ⇑ I-Chen Kimberly Chen a,b, , Brad Griesenauer a, Yuen-Tsu Nicco Yu b, Gregory J. Velicer a,b a Department of Biology, Indiana University, Bloomington, IN 47405, USA b Institute of Integrative Biology (IBZ), ETH Zurich, CH-8092 Zurich, Switzerland article info abstract Article history: In animals and plants, non-coding small RNAs regulate the expression of many genes at the post-tran- Received 26 August 2013 scriptional level. Recently, many non-coding small RNAs (sRNAs) have also been found to regulate a vari- Revised 30 December 2013 ety of important biological processes in bacteria, including social traits, but little is known about the Accepted 2 January 2014 phylogenetic or mechanistic origins of such bacterial sRNAs. Here we propose a phylogenetic origin of Available online 10 January 2014 the myxobacterial sRNA Pxr, which negatively regulates the initiation of fruiting body development in Myxococcus xanthus as a function of nutrient level, and also examine its diversification within the Keywords: Myxococcocales order. Homologs of pxr were found throughout the Cystobacterineae suborder (with a Bacterial development few possible losses) but not outside this clade, suggesting a single origin of the Pxr regulatory system Multicellularity Myxobacteria in the basal Cystobacterineae lineage. Rates of pxr sequence evolution varied greatly across Cystobacte- Non-coding small RNAs rineae sub-clades in a manner not predicted by overall genome divergence. -
The Existence and Diversity of Myxobacteria in Lake Mud A
bs_bs_banner Environmental Microbiology Reports (2012) doi:10.1111/j.1758-2229.2012.00373.x The existence and diversity of myxobacteria in lake mud – a previously unexplored myxobacteria habitat Shu-guang Li,† Xiu-wen Zhou,† Peng-fei Li, Kui Han, et al., 2003). Using fruiting body-dependent techniques, Wei Li, Zhi-feng Li, Zhi-hong Wu and Yue-zhong Li* myxobacteria have been isolated from various soil-related State Key Laboratory of Microbial Technology, School of samples, including soil, rotting wood, bark from dead or Life Science, Shandong University, Jinan 250100, living trees and dung of herbivorous mammals, but rarely China. from aquatic environments (Reichenbach, 1999; Dawid, 2000). Accordingly, myxobacteria are widely recognized as typical soil bacteria (Reichenbach, 1999). Recently, Summary halophilic (Iizuka et al., 1998; 2003; Fudou et al., 2002) Myxobacteria are widely distributed in soil and and halotolerant (Li et al., 2002) myxobacterial strains oceanic sediment with a phylogeographic separation have been isolated from coastal areas. They have been at high levels of classification. However, it is unclear shown to exhibit certain characteristics that differ from whether freshwater environments, from which there their soil-dwelling relatives (Zhang et al., 2005; Wang has been no isolation report of myxobacteria since et al., 2007a). Molecular surveys have indicated that 1981, are habitats for myxobacteria. In this study, we myxobacteria-related 16S rRNA gene sequences are also investigated the presence of myxobacteria in lake widely distributed in marine sediments at different depths mud using a two-step strategy. First, we constructed and sites, but they are distantly related to those of soil two universal bacterial libraries from the V3–V4 (V34) myxobacteria (Jiang et al., 2010; Brinkhoff et al., 2012). -
Evolution of Developmental Gene Regulation in the Myxobacteria
EVOLUTION OF DEVELOPMENTAL GENE REGULATION IN THE MYXOBACTERIA I-Chen Chen Submitted to the faculty of the University Graduate School in partial fulfillment of the requirements for the degree Doctor of Philosophy in the Department of Biology, Indiana University July 2015 Accepted by the Graduate Faculty, Indiana University, in partial fulfillment of the requirements for the degree of Doctor of Philosophy. Doctoral Committee Gregory J. Velicer, Ph.D. Patricia L. Foster, Ph.D. Jeffrey D. Palmer, Ph.D. Rudolf A. Raff, Ph.D. Michael J. Wade, Ph.D. Yuen-Tsu Nicco Yu, Ph.D. June 10th, 2015 ii Copyright © 2015 I-Chen Chen All Rights Reserved iii ACKNOWLEDGEMENTS First of all, I would like to thank my two advisors Greg Velicer and Nicco Yu for their support and relentless encouragement during every stage in my PhD. I will be forever grateful for their guidance and the synergy they provided. In addition, I would like to thank Nicco for helping me with my transitions to Bloomington and Zürich and turning me into a cat person. I would also like to thank my committee members, Jeff Palmer and Mike Wade, for their guidance, encouragement and critique on my research through my PhD, and Pat Foster and Rudy Raff for kindly joining the committee later on. I am indebted to many graduate students, postdocs and faculty in the Biology Department at Indiana University, and in the Theoretical Biology, Experimental Ecology, Molecular Microbial Ecology groups at ETH Zürich for the supportive and stimulating research environments they provided. It was a lot of fun working on the myxobacteria in the Velicer lab. -
Biology 1015 General Biology Lab Taxonomy Handout
Biology 1015 General Biology Lab Taxonomy Handout Section 1: Introduction Taxonomy is the branch of science concerned with classification of organisms. This involves defining groups of biological organisms on the basis of shared characteristics and giving names to those groups. Something that you will learn quickly is there is a lot of uncertainty and debate when it comes to taxonomy. It is important to remember that the system of classification is binomial. This means that the species name is made up of two names: one is the genus name and the other the specific epithet. These names are preferably italicized, or underlined. The scientific name for the human species is Homo sapiens. Homo is the genus name and sapiens is the trivial name meaning wise. For green beans or pinto beans, the scientific name is Phaseolus vulgaris where Phaseolus is the genus for beans and vulgaris means common. Sugar maple is Acer saccharum (saccharum means sugar or sweet), and bread or brewer's yeast is Saccharomyces cerevisiae (the fungus myces that uses sugar saccharum for making beer cerevisio). In taxonomy, we frequently use dichotomous keys. A dichotomous key is a tool for identifying organisms based on a series of choices between alternative characters. Taxonomy has been called "the world's oldest profession", and has likely been taking place as long as mankind has been able to communicate (Adam and Eve?). Over the years, taxonomy has changed. For example, Carl Linnaeus the most renowned taxonomist ever, established three kingdoms, namely Regnum Animale, Regnum Vegetabile and Regnum Lapideum (the Animal, Vegetable and Mineral Kingdoms, respectively). -
Correlating Chemical Diversity with Taxonomic Distance for Discovery of Natural Products in Myxobacteria
ARTICLE DOI: 10.1038/s41467-018-03184-1 OPEN Correlating chemical diversity with taxonomic distance for discovery of natural products in myxobacteria Thomas Hoffmann1,2, Daniel Krug 1,2, Nisa Bozkurt1, Srikanth Duddela1, Rolf Jansen3, Ronald Garcia1,2, Klaus Gerth3, Heinrich Steinmetz3 & Rolf Müller 1,2 1234567890():,; Some bacterial clades are important sources of novel bioactive natural products. Estimating the magnitude of chemical diversity available from such a resource is complicated by issues including cultivability, isolation bias and limited analytical data sets. Here we perform a systematic metabolite survey of ~2300 bacterial strains of the order Myxococcales, a well- established source of natural products, using mass spectrometry. Our analysis encompasses both known and previously unidentified metabolites detected under laboratory cultivation conditions, thereby enabling large-scale comparison of production profiles in relation to myxobacterial taxonomy. We find a correlation between taxonomic distance and the pro- duction of distinct secondary metabolite families, further supporting the idea that the chances of discovering novel metabolites are greater by examining strains from new genera rather than additional representatives within the same genus. In addition, we report the discovery and structure elucidation of rowithocin, a myxobacterial secondary metabolite featuring an uncommon phosphorylated polyketide scaffold. 1 Helmholtz Institute for Pharmaceutical Research Saarland (HIPS), Department of Microbial Natural Products, Helmholtz Centre for Infection Research and Department of Pharmaceutical Biotechnology, Saarland University, Campus E8.1, 66123 Saarbrücken, Germany. 2 German Centre for Infection Research (DZIF), Partner Site Hannover-Braunschweig, 38124 Braunschweig, Germany. 3 Helmholtz Centre for Infection Research (HZI), Department of Microbial Drugs, 38124 Braunschweig, Germany. Thomas Hoffmann and Daniel Krug contributed equally to this work.