The Composition of Cosmic Rays at the Knee
Total Page:16
File Type:pdf, Size:1020Kb
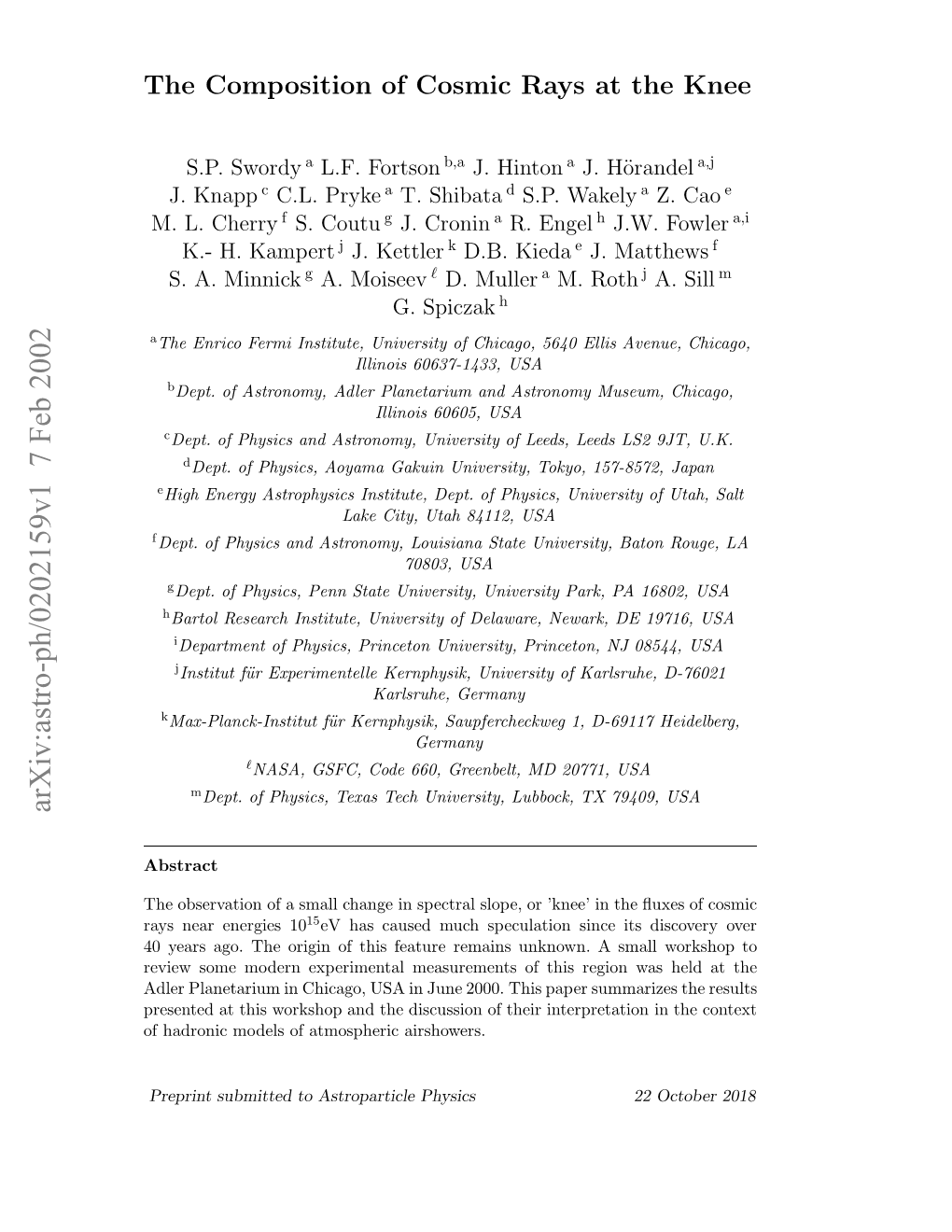
Load more
Recommended publications
-
Dissertation Zur Erlangung Des Akademischen Grades Eines Doktors Der Naturwissenschaften
System Tests, Initial Operation and First Data of the AMIGA Muon Detector for the Pierre Auger Observatory Dissertation zur Erlangung des akademischen Grades eines Doktors der Naturwissenschaften vorgelegt von Master of Science Michael Pontz, geboren am 17. September 1982 in Mainz, dem Department Physik der Naturwissenschaftlich-Technischen Fakultät der Universität Siegen Siegen, Dezember 2012 Berichte aus der Physik Michael Pontz System Tests, Initial Operation and First Data of the AMIGA Muon Detector for the Pierre Auger Observatory Shaker Verlag Aachen 2013 Bibliographic information published by the Deutsche Nationalbibliothek The Deutsche Nationalbibliothek lists this publication in the Deutsche Nationalbibliografie; detailed bibliographic data are available in the Internet at http://dnb.d-nb.de. Zugl.: Siegen, Univ., Diss., 2013 Copyright Shaker Verlag 2013 All rights reserved. No part of this publication may be reproduced, stored in a retrieval system, or transmitted, in any form or by any means, electronic, mechanical, photocopying, recording or otherwise, without the prior permission of the publishers. Printed in Germany. ISBN 978-3-8440-1758-8 ISSN 0945-0963 Shaker Verlag GmbH • P.O. BOX 101818 • D-52018 Aachen Phone: 0049/2407/9596-0 • Telefax: 0049/2407/9596-9 Internet: www.shaker.de • e-mail: [email protected] Abstract Investigating the energy region between 1017 eV and 4 1018 eV for primary cosmic particles will lead to a deeper understanding of the origin× of cosmic rays. Effects of the transition from galactic to extragalactic origin are expected to be visible in this region. The knowledge of the composition of cosmic rays strongly depends on the hadronic interaction models, which are applied in the air shower reconstruction. -
A Measurement of the Ultra-High Energy Cosmic Ray Flux with the Hires Fadc Detector
A MEASUREMENT OF THE ULTRA-HIGH ENERGY COSMIC RAY FLUX WITH THE HIRES FADC DETECTOR BY ANDREAS ZECH A dissertation submitted to the Graduate School—New Brunswick Rutgers, The State University of New Jersey in partial fulfillment of the requirements for the degree of Doctor of Philosophy Graduate Program in Physics and Astronomy Written under the direction of Prof. Gordon Thomson and approved by New Brunswick, New Jersey October, 2004 °c 2004 Andreas Zech ALL RIGHTS RESERVED ABSTRACT OF THE DISSERTATION A Measurement of the Ultra-High Energy Cosmic Ray Flux with the HiRes FADC Detector by Andreas Zech Dissertation Director: Prof. Gordon Thomson We have measured the ultra-high energy cosmic ray flux with the newer one of the two detectors of the High Resolution Fly’s Eye experiment (HiRes) in monocular mode. An outline of the HiRes experiment is given here, followed by a description of the trigger and Flash ADC electronics of the HiRes-2 detector. The computer simulation of the experiment, which is needed for resolution studies and the calculation of the detector acceptance, is presented in detail. Different characteristics of the simulated events are compared to real data to test the performance of the Monte Carlo simulation. The calculation of the energy spectrum is described, together with studies of systematic uncertainties due to the cosmic ray composition and aerosol content of the atmosphere that are assumed in the simulation. Data collected with the HiRes-2 detector between December 1999 and September 2001 are included in the energy spectrum presented here. We compare our result with previous measurements by other experiments. -
Ultra High Energy Cosmic Ray Research with CASA-MIA
Ultra High Energy Cosmic Ray Research with CASA-MIA Rene A. Ong Department of Physics and Astronomy University of California, Los Angeles Los Angeles, CA 90095-1547, USA Abstract Ultra high energy (UHE) cosmic rays are particles reaching Earth that have energies greater than 1014 eV [1]. These particles are produced in astrophysical sources that are sites of extreme particle acceleration but because they are largely charged, they bend in the mag- netic field of the Galaxy and their directional information is scrambled by the time they reach Earth. Thus, even after many years of research, the origin of these particles remains a profound mystery. Starting in 1985, Jim Cronin developed the idea for a large air shower array to search for and detect point sources of gamma rays at these energies. The idea was to build a power- ful enough detector to definitively establish the existence of gamma-ray point sources and to hence determine the origin of the ultra high energy cosmic rays. This idea became the Chicago Air Shower Array – MIchigan muon Array (CASA-MIA). During the decade be- tween 1987 and 1997, CASA-MIA was constructed and was successfully operated. It re- mains the most sensitive cosmic-ray observatory ever built at these energies. This talk de- scribes the CASA-MIA experiment: its scientific motivation, its development, construction and operation, and its scientific results and legacy. 1 Scientific Motivation for CASA-MIA Cosmic rays were discovered in 1912 by Victor Hess during balloon experiments carried out in Austria. Hess flew in an open-air balloon to altitudes of 5 km and discovered an increasing ionization of the air with increasing altitude rather than a decreasing one [2]. -
Astroparticle Physics Plays a Very Important Role
DFUB 13/2002 Bologna, 05/11/02 ASTROPARTICLE PHYSICS G. GIACOMELLI AND M. SIOLI Dipartimento di Fisica dell’Universit`adi Bologna and INFN, Sezione di Bologna Viale C. Berti Pichat 6/2, I-40127, Bologna, Italy e–mail: [email protected], [email protected] Lectures at the 6th Constantine School on ”Weak and Strong Interactions Phenomenology” Mentouri University, Constantine, Algeria , 6-12 April 2002 Abstract. The main issues in non-accelerator astroparticle physics are reviewed and discussed. A short description is given of the experimental methods, of many experiments and of their experimental results. 1. Introduction The present Standard Model (SM) of particle physics, which includes the Glashow-Weinberg-Salam theory of Electroweak Interactions (EW) and Quantum Chromodynamics (QCD) for the Strong Interaction, explains quite well all available experimental results. The theory had surprising con- firmations from the precision measurements performed at LEP [1.1, 1.2]. There is one important particle still missing in the SM: the Higgs Boson. Hints may have been obtained at LEP and lower mass limits established at arXiv:hep-ex/0211035v1 13 Nov 2002 ∼ 114 GeV. On the other hand few physicists believe that the SM is the ultimate theory, because it contains too many free parameters, there is a prolifera- tion of quarks and leptons and because it seems unthinkable that there is no further unification with the strong interaction and eventually with the gravitational interaction. Thus most physicists consider the present Stan- dard Model of particle physics as a low energy limit of a more fundamental theory, which should reveal itself at higher energies. -
Experimental Installations
Appendix A Experimental Installations A.1 EAS Arrays and Cosmic Ray Ground Facilities A.1.1 Lists of Array and Facility Sites In the following we present a list of air shower arrays of the past and present (Table A.1). The altitudes of the arrays are listed together with the corresponding atmospheric depths. The latter are in most cases the vertical atmospheric depths, i.e., the average vertical overburden [g cm−2]. In some cases, however, authors may specify different values for the overburden in different publications for the same array. The reason for these discrepancies is that in some cases authors consider the slant depth for the average near vertical shower, e.g., ∼15◦, as compared to the depth for perfectly vertical showers, that are rather rare exceptions in practice. Some table entries with similar altitudes suggest contradicting altitude-overburden relations, but these are explained by differing average atmospheric and meteorological conditions (see Sect. B.3). Figure A.1, which is a reproduction from a web page of the Yakutsk group, shows the aperture in units of square kilometer-steradian [km2 sr] of the largest air shower arrays of the past and present, and of arrays and detector systems currently under construction or in the planing phase. In Table A.2 we list emulsion chamber sites, and in Table A.3 the threshold energies of the muon detectors of some of the arrays listed in Table A.1. Note that for some arrays different threshold energies were used simultaneously, in others different thresholds at different times. Table A.4 is a partial list of major gamma ray air Cherenkov detectors of past and present. -
Extensive Air Shower Radio Detection: Recent Results and Outlook
Extensive Air Shower Radio Detection: Recent Results and Outlook 1 Jonathan L. Rosner and Denis A. Suprun Enrico Fermi Institute and Department of Physics University of Chicago, Chicago, IL 60637 USA Abstract. A prototype system for detecting radio pulses associated with extensive cosmic ray air showers is described. Sensitivity is compared with that in previous experiments, and lessons are noted for future studies. I INTRODUCTION The observation of the radio-frequency (RF) pulse associated with extensive air showers of cosmic rays has had a long and checkered history. In the present report we describe an attempt to observe such a pulse in conjunction with the Chicago Air Shower Array (CASA) and Michigan Muon Array (MIA) at Dugway, Utah. Only upper limits on a signal have been obtained at present, though we are still processing data and establishing calibrations. arXiv:astro-ph/0101089v4 14 Apr 2003 In Section 2 we review the motivation and history of RF pulse detection. Sec- tion 3 is devoted to the work at CASA/MIA, while Section 4 deals with some future possibilities, including ones associated with the planned Pierre Auger observatory. We conclude in Section 5. This report is an abbreviated version of a longer one [1]. II MOTIVATION AND HISTORY A Auxiliary information on shower Present methods for the detection of an extensive air shower of cosmic rays leave gaps in our information. The height above ground at which showers develop cannot be provided by ground arrays, though stereo detection by air fluorescence detectors is useful. Composition of the primary particles, another unknown, is correlated 1) Invited talk presented by J. -
By David Clarke Prosser B.Sc. Submitted in Accordance with The
A SEARCH FOR GAMMA-RAY EMISSION AT ENERGIES GREATER THAN 1014eV FROM CYGNUS X-3 AND EIGHT OlHER CANDIDATE SOURCES by David Clarke-- Prosser B.Sc. Submitted in accordance with the requirements for the degree of Doctor of Philosophy The candidate confinns that the work submitted is his own and that appropriate credit has been given where reference has been made to the works of others The University of Leeds December 1991 Department of Physics i ABSTRACT The discovery of cosmic rays with energies greater than 1014e V has posed a question that has not, as yet, been answered: where are particles accelerated to such high energies? A possible answer to this question came in 1983 with the claim made by Samorski and Stamm of an excess number of cosmic rays from the direction of Cygnus X-3. This result was then conf1l1lled by Lloyd-Evans et al. (1983). The excess was taken to be gamma-rays as the galactic magnetic fields result in charged particles being greatly deflected. These claims led to the birth of PeV gamma-ray astronomy and the building of numerous instruments designed to search for point sources of Pe V gamma-ray emission. One such instrument was the GREX extensive air shower array built at Haverah Park which began collecting data in March 1986. This thesis describes the GREX array and the methods of analysis used to Dl reconstruct the size and arrival direction"the _incident cosmic rays from the detected air showers. The methods used to search for potential point sources are then described. -
Acronyms and Abbreviations
EUSO Instrument DOCUMENT: EUSO-PI-REP-002 ISSUE: - Report on the Phase A Study REVISION: - DATE: - JULY 2003 PAGE: 1/12 Acronyms and Abbreviations A ASI Agenzia Spaziale Italiana, Italy AC Adjudication Committee ASIC Application Specific Integrated Circuit AC Alternate Current ASQC American Society of Quality Control ACCESS Advanced Cosmic Ray Composition ASW Application Software Experiment on Space Station ATIC Advanced Thin Ionization Calorimeter ACRO Pierre Auger Cosmic Ray Observatory ATLID Atmospheric LIDar (PAO) ATP Authority to Proceed AD Applicable Document ATP Authorization to Proceed AD Architectural Design AU Astronomical Unit ADC Analog to Digital Converter AVS AVionicS ADD Architectural Design Document AW AirWatch ADM The Atmospheric Dynamics Mission AWG American Wire Gauge AFEE Analog Front End Electronics AWG Astronomy Working Group (ESA) AGASA Akeno Giant Air Shower Array AWS AirWatch from Space AGN Active Galactic Nuclei B AI Action Item BABY Background BYpass AI Analog Input BAD Broadcast Ancillary Data AIRES AIR-shower Extended Simulations S/W BASIS Burst arc second Imaging and Spectroscopy AIS Automated Information system BATSE Burst and Transient Spectroscopy AIT Assembly Integration and Test Experiment AIV Assembly Integration and Verification BC Bus Controller AIV/T Assembly, Integration and Verification & BCD Binary Coded Decimal Test BD Block Diagram ALS Alenia Spazio BDR Baseline Design Review AM Atmosphere Monitoring BeppoSAX X-ray astronomy Satellite AME Analog Macrocell Electronics BF Bleeder Factor AMS Alpha -
Constraints on Gamma-Ray Emission from the Galactic Plane at 300
Submitted to The Astrophysical Journal EFI Preprint 96-43 Constraints on Gamma-ray Emission from the Galactic Plane at 300 TeV A. Borione,1 M.A. Catanese,2,4 M.C. Chantell,1 C.E. Covault,1 J.W. Cronin,1 B.E. Fick,1 L.F. Fortson,1 J. Fowler,1 M.A.K. Glasmacher,2 K.D. Green,1 D.B. Kieda,3 J. Matthews,2 B.J. Newport,1 D. Nitz,2 R.A. Ong,1 S. Oser,1 D. Sinclair,2 J.C. van der Velde2 ABSTRACT We describe a new search for diffuse ultrahigh energy gamma-ray emission associated with molecular clouds in the galactic disk. The Chicago Air Shower Array (CASA), operating in coincidence with the Michigan muon array (MIA), has recorded over 2.2 109 air showers from × April 4, 1990 to October 7, 1995. We search for gamma rays based upon the muon content of air showers arriving from the direction of the galactic plane. We find no significant evidence for diffuse gamma-ray emission, and we set an upper limit on the ratio of gamma rays to normal hadronic cosmic rays at less than 2.4 10−5 at 310 TeV (90% confidence limit) from the galactic ◦ ◦ ◦ × ◦ plane region: (50 <ℓII < 200 ; 5 <bII < 5 ). This limit places a strong constraint on models − for emission from molecular clouds in the galaxy. We rule out significant spectral hardening in the outer galaxy, and conclude that emission from the plane at these energies is likely to be dominated by the decay of neutral pions resulting from cosmic rays interactions with passive target gas molecules. -
James Pinfold Reports on the Large Number of Projects That Are Forging
CCEMaySCHOOLS19-22 13/4/06 10:34 Page 19 COSMIC RAYS High schools focus on the extreme universe James Pinfold reports on the large number of projects that are forging a connection between research in ultra-high-energy cosmic rays and practical scientific experience in schools. Installing the first educational cosmic-ray array on the roof of Detectors from the Chicago Air Shower Array have become a Archbishop O’Leary High School in Edmonton, Alberta, on garden feature at the Chaminade Middle School, where they 23 February 1998. The array is part of the ALTA experiment. form part of the California High School Cosmic Ray Observatory. On 15 October 1991 the highest-energy cosmic-ray particle ever mea- rapidly lose their energy in collisions with the 2.7 K cosmic-microwave sured struck Earth’s atmosphere tens of kilometres above the Utah background radiation from the Big Bang – the Greisen–Zatsepin– Desert. Colliding with a nucleus, it lit up the night for an instant and Kuzmin limit. While many explanations have been proposed, experi- then was gone. The Fly’s Eye detector at the Dugway Proving Grounds ments have so far failed to decipher a clear message from these in Utah captured the trail of light emitted as the cascade of secondary highly energetic messengers, and the existence of the OMG events particles created in the collision made the atmosphere fluoresce. The has become a profound puzzle. Now a new eye on these ultra-high- Fly’s Eye researchers measured the energy of the unusual ultra-high- energy events has come into focus, based on the great plain of the energy cosmic-ray event – dubbed the “Oh-My-God (OMG) event” – at Pampa Amarilla in western Argentina. -
PHY418 Particle Astrophysics Susan Cartwright
PHY418 Particle Astrophysics Susan Cartwright Contents 1 Introduction 7 1.1 Whatisparticleastrophysics?. 7 1.2 Early-universecosmology . 8 1.2.1 Inflation............................ 9 1.2.2 Baryogenesis ......................... 11 1.3 Thephysicsofdarkenergy . 17 1.4 High-energyprocessesinastrophysics. 20 1.4.1 The non-thermal universe . 21 1.4.2 Detectiontechniques . 22 1.5 Neutrinos ............................... 25 1.5.1 Neutrinosincosmology . 25 1.5.2 Solarneutrinos ........................ 26 1.5.3 Supernovaneutrinos . 26 1.5.4 Atmospheric neutrinos . 28 1.5.5 High-energyneutrinos . 28 1.6 Darkmatter.............................. 29 1.6.1 Astrophysical and cosmological evidence for dark matter . 29 1.6.2 Darkmattercandidates . 30 1.6.3 Detectionofdarkmatter . 34 1.7 Summary ............................... 40 1.8 QuestionsandProblems . 40 2 Astrophysical Accelerators: The Observational Evidence 43 2.1 Introduction.............................. 43 2.2 Cosmicrays.............................. 44 2.2.1 Abriefhistory ........................ 44 2.2.2 Detectionofcosmicrays . 45 2.2.3 Observedproperties . 56 2.3 Radioemission ............................ 69 2.3.1 Theradiosky......................... 69 2.3.2 Radio emission mechanisms . 71 2.3.3 Electromagnetic radiation from an accelerated charge . 73 2.3.4 Bremsstrahlung. 76 2.3.5 Synchrotron radiation . 80 2.3.6 Selfabsorption ........................ 86 2.3.7 Summary ........................... 88 2.4 Highenergyphotons ......................... 89 2.4.1 High energy photons and particle astrophysics . 89 2.4.2 Mechanisms of high-energy photon emission . 89 2.4.3 X-rays............................. 94 2.4.4 Soft γ-rays .......................... 97 2.4.5 Intermediate energy γ-rays .................103 3 4 2.4.6 High-energy γ-rays......................106 2.5 High-energyneutrinos . .118 2.5.1 Neutrino production and the Waxman–Bahcall bound . -
Preliminary Results from the Chicago Air Shower Array and the Michigan Muon Array*
PRELIMINARY RESULTS FROM THE CHICAGO AIR SHOWER ARRAY AND THE MICHIGAN MUON ARRAY* H.A. Krimm, J.W. Cronin, B.E. Fick, K.G. Gibbs, N.C. Mascarenhas, T.A. McKay, D. Mfiller, B.J. Newport, R.A. Ong, L.J Rosenberg, M.E. Wiedenbeck The Enrico Fermi Institute, The University of Chicago, Chicago, IL 60637 U.S.A. K.D. Green, J. Matthews, D. Nitz, D. Sinclair, J.C. van der Velde Department of Physics, The University of Michigan, Ann Arbor, MI 48109 U.S.A. ABSTRACT The Chicago Air Shower Array (CASA) is a large area surface array designed to de- tect extensive air showers (EAS) produced by primaries with energy ~100 TeV. It operates in coincidence with the underground Michigan Muon Array (MIA). Preliminary results are pre- sented from a search for steady emission and daily emission from three astrophysical sources: Cygnus X-3, Hercules X-l, and the Crab nebula and pulsar. There is no evidence for a signifi- cant signal from any of these sources in the 1989 data. I. INTRODUCTION The Chicago Air Shower Array and the Michigan Muon Array are located at Dugway, Utah, U.S.A. (40.20 N, 112.8° W) at an atmospheric depth of 870 g • cm -~. Detailed descrip- tions of CASAt,2 and MIAs can be found elsewhere. From 1 February 1989 to 30 November 1989, CASA operated with 49 detector stations, each containing 1.5 m 2 of plastic scintillator, arranged on a square grid of total area 8100 m 2 . MIA consisted of 512 buried counters of area 2.5 m 2 distributed in eight widely separated patches.