On the Recirculation of Ammonia-Lithium Nitrate in Adiabatic Absorbers for Chillers R
Total Page:16
File Type:pdf, Size:1020Kb
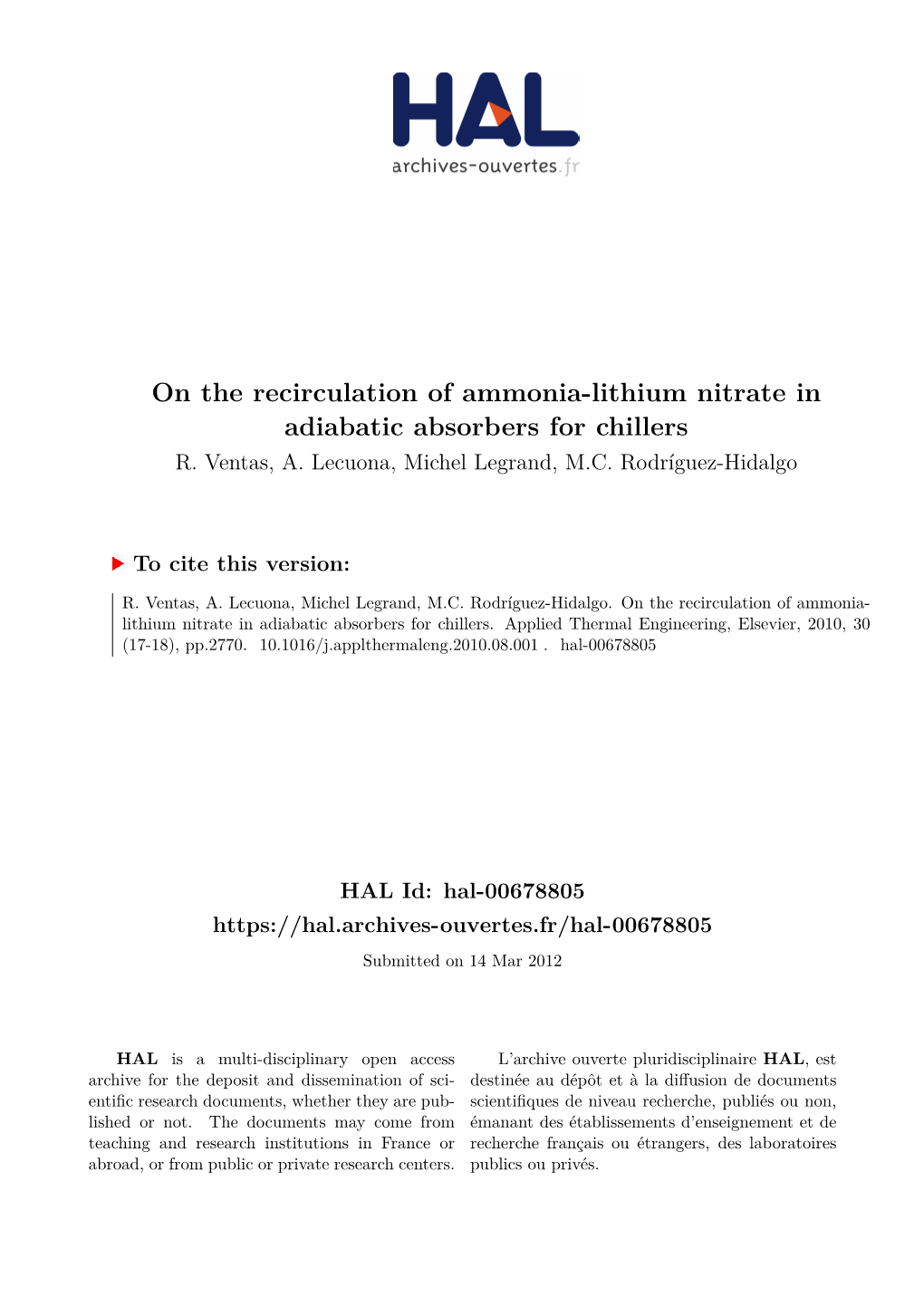
Load more
Recommended publications
-
CCXL1.- the Electrochemistry of Solutions in Acetone. Part I
View Article Online / Journal Homepage / Table of Contents for this issue 2 I38 ROSHDESTWENSKY AND LEWlS THE CCXL1.- The Electrochemistry of Solutions in Acetone. Part I. By ALEXANDERROSHDESTWENSEY and WILLIAM CUDMORE MCCULLAGHLEWIS. WHILSTa considerable amount of attention has been directed to the electrochemical behaviour of methyl and ethyl alcohols, rela- tively few measurements are recorded in the caw of solutions in acetone. The present paper contains the resulk of an investigation undertaken to supply to a certain extent this deficiency. The solutes employed were lithium nitrate and silver nitrate, the latter being examined in greater detail. Before giving our own meamrements, it is necessary to indicate briefly some of the results obtained by other observers which bear directly on the results obtained by us.* As regards the electrolysis of saturated silver nitrate solutions in acetone, Kahlenberg (J. physicd C‘Aem., 1900,4, 349) found that the metal was precipitated in a coherent form, but “the solution conducts so poorly ” that it was impossible to verify Faxaday’s law. In the same paper Kahlenberg gives some results of electromotive force measurements of Ag i AgNO, concentration cells in pyridine and acetonitrile respectively, with regard to which he concludes that the Nernst expressions are inapplicable. Kahlenberg asumes that the liquid/liquid potential difference is negligible, which, in the case of acetone at least, we shall show later is not the case. The specific conductivity of acetone itself has been meamred by Dutoit and Levier (J. Chim. phys., 1905, 3,435), the value obtained Published on 01 January 1911. Downloaded by Freie Universitaet Berlin 09/04/2018 17:53:03. -
LISTA PUBLIKACJI 2009 LIST of PUBLICATIONS
Instytut Niskich Temperatur i Badań Strukturalnych PAN Wrocław, 2011.12 31 LISTA PUBLIKACJI 2009 LIST of PUBLICATIONS KSIĄŻKI, MONOGRAFIE i ARTYKUŁY PRZEGLĄDOWE Books, Monographs & Reviews 1. R. Troć, 6.β Actinide monochalcogenides. In: Landolt–Börnstein Numerical Data and Functional Relationship in Science and Technology, New Series, ed. by W.Martienssen, Group III: Condensed Matter, Vol. 27: Magnetic Properties of Non-Metallic Inorganic Compounds Based on Transition Elements, ed. by H.P.J. Wijn, Subvol. B Pnictides and Chalcogenides III (Berlin: Springer-Vg 2009) Pt 6β, X + 574 pp. ARTYKUŁY W CZASOPISMACH NAUKOWYCH Articles in Scientific Journals 2. L.C.Alves, M.M.M.Rubinger, R.H.Lindemann, G.J.Perpétuo, J. Janczak, L.D.L.Miranda, L.Zambolim, M.R.L.Oliveira, Syntheses, Crystal Structure, Spectroscopic Characterization and Antifungal Activity of New N -R-Sulfonyldithiocarbimate Metal Complexes. J. Inorg. Biochem. 103 7 (2009) 1045–53. [DOI] 3. R.Acevedo, A.Soto-Bubert, M.E.G.Valerio, W. Stręk, On the Theory of Interaction Potentials in Ionic Crystals: An Application to the Thermodynamics of Lanthanide Type Crystals. Asian J. Spectrosc. 13 1 (2009) 43–65. 4. L.G.Akselrud, I.A.Ivashchenko, O.F.Zmiy, I.D.Olekseyuk, J. Stępień-Damm, Description of Concentration Polytypism in Cd1−xCuxIn2Se4 by Commensurately Modulated Structures. Chem. Met. Alloys 2 1/2 (2009) 108–14. 5. Tran Kim Anh, Dinh Xuan Loc, Lam thi Kieu Giang, W. Stręk, Le Quoc Minh, Preparation, Optical Properties of ZnO, ZnO : Al Nanorods and Y(OH)3 : Eu Nanotube. J. Phys. Conf. Ser. 146 (2009) 012001 (5). [DOI] II Kraj.Konf. -
Techno-Economic Forecasts of Lithium Nitrates for Thermal Storage Systems
Article Techno-Economic Forecasts of Lithium Nitrates for Thermal Storage Systems Macarena Montané 1,*, Gustavo Cáceres 1, Mauricio Villena 2 and Raúl O’Ryan 1 1 Facultad de Ingeniería y Ciencias, Universidad Adolfo Ibáñez, Avenida Diagonal Las Torres 2640, Peñalolén, Santiago 7941169, Chile; [email protected] (G.C.); [email protected] (R.O.) 2 Escuela de Negocios, Universidad Adolfo Ibáñez, Avenida Diagonal Las Torres 2640, Peñalolén, Santiago 7941169, Chile; [email protected] * Corresponding author: [email protected]; Tel.: +56-2-2331-1416 Academic Editor: Manfred Max Bergman Received: 24 March 2017; Accepted: 8 May 2017; Published: 12 May 2017 Abstract: Thermal energy storage systems (TES) are a key component of concentrated solar power (CSP) plants that generally use a NaNO3/KNO3 mixture also known as solar salt as a thermal storage material. Improvements in TES materials are important to lower CSP costs, increase energy efficiency and competitiveness with other technologies. A novel alternative examined in this paper is the use of salt mixtures with lithium nitrate that help to reduce the salt’s melting point and improve thermal capacity. This in turn allows the volume of materials required to be reduced. Based on data for commercial plants and the expected evolution of the lithium market, the technical and economic prospects for this alternative are evaluated considering recent developments of Lithium Nitrates and the uncertain future prices of lithium. Through a levelized cost of energy (LCOE) analysis it is concluded that some of the mixtures could allow a reduction in the costs of CSP plants, improving their competitiveness. -
Effects of Lithium Nitrate Admixture on Early Age
EFFECTS OF LITHIUM NITRATE ADMIXTURE ON EARLY AGE CONCRETE BEHAVIOR A Thesis Presented to the Academic Faculty By Marcus J. Millard In Partial Fulfillment of the Requirements for the Degree Master of Science in Civil and Environmental Engineering Georgia Institute of Technology August 2006 EFFECTS OF LITHIUM NITRATE ADMIXTURE ON EARLY AGE CONCRETE BEHAVIOR Approved by: Dr. Kimberly E. Kurtis, Advisor School of Civil and Environmental Engineering Georgia Institute of Technology Dr. Lawrence F. Kahn, Professor School of Civil and Environmental Engineering Georgia Institute of Technology Dr. James S. Lai, Professor Emeritus School of Civil and Environmental Engineering Georgia Institute of Technology Date Approved: July 10, 2006 TABLE OF CONTENTS LIST OF TABLES v LIST OF TABLES vi SUMMARY x I. INTRODUCTION 1 II. LITERATURE REVIEW 6 2.1 ASR Reactions and Mechanisms of Damage 6 2.2 Prevention of ASR Damage in New Concrete Construction 8 2.2.1 Materials Selection for ASR mitigation 8 2.2.2 Lithium-containing admixtures for ASR mitigation 9 2.3 Effects of Lithium Admixtures on Early Age Behavior 11 2.3.1 Concrete Pore Solution and Hydration Product Chemistry 12 2.3.2 Workability 13 2.3.3 Setting Time 14 2.3.4 Shrinkage 16 2.3.5 Air Content and Unit Weight 16 2.3.6 Strength 18 III. MATERIALS AND EXPERIMENTAL METHODS 23 3.1 Materials 23 3.1.1 Concrete Materials 24 3.1.2 Lithium 27 3.2 Experimental Methods 29 3.2.1 Isothermal Calorimetry 32 3.2.2 Rheology and Slump 33 3.2.3 Bleeding 36 3.2.4 Vicat Time of Setting 37 3.2.5 Chemical Shrinkage 40 3.2.6 Autogenous Shrinkage 43 3.2.7 Free Shrinkage 45 3.2.8 Restrained Shrinkage 47 3.2.9 Strength 49 IV. -
Thermodynamic Properties of Molten Nitrate Salts
THERMODYNAMIC PROPERTIES OF MOLTEN NITRATE SALTS Joseph G. Cordaro1, Alan M. Kruizenga2, Rachel Altmaier2, Matthew Sampson2, April Nissen2 1 Sandia National Laboratories: Senior member, Technical Staff, PhD. PO Box 969, Livermore, CA, USA, 94551, Phone: +1 (925) 294-2351, Email: [email protected] 2 Sandia National Laboratories: PO Box 969, Livermore, CA, USA, 94551 Abstract Molten salts are being employed throughout the world as heat transfer fluids and phase change materials for concentrated solar power (CSP) production and storage. In order to design large production facilities, accurate physical and thermodynamic properties of molten salts must be known. Required data include a) melting point; b) viscosity; c) apparent heat of fusion; d) thermal conductivity; e) heat capacity; f) density; g) volumetric expansion; and h) vapor pressure. Data are available for many pure components which make up molten salt mixtures. However, there are some missing or inconsistent numbers in the literature, especially for materials at elevated temperatures. This paper summarizes our most current thermodynamic measurements for some pure salts and mixtures which are of interest as heat transfer fluids and phase change materials (PCM). Specifically, data on the apparent heat of fusion (ΔHfus) and heat capacity (Cp) for a family of nitrate and nitrite salts are reported. These data are critical for estimating costs associated with parabolic trough systems, central receiver towers, and thermal storage tanks. Keywords: Nitrate, Molten Salts, Heat Capacity, heat of Fusion 1. Introduction Our current work focuses on expanding the database of thermophysical properties of alkali and alkaline earth cations with nitrate or nitrite anions. Previous reports from our group discuss low-melting salts containing lithium, sodium, potassium and calcium cations with nitrate and nitrite anions. -
The Use of Lithium to Prevent Or Mitigate Alkali-Silica Reaction in Concrete Pavements and Structures
The Use of Lithium to Prevent or Mitigate Alkali-Silica Reaction in Concrete Pavements and Structures PUBLIcatION NO. FHWA-HRT-06-133 MARCH 2007 Research, Development, and Technology Turner-Fairbank Highway Research Center 6300 Georgetown Pike McLean, VA 22101-2296 Foreword Progress is being made in efforts to combat alkali-silica reaction in portland cement concrete structures—both new and existing. This facts book provides a brief overview of laboratory and field research performed that focuses on the use of lithium compounds as either an admixture in new concrete or as a treatment of existing structures. This document is intended to provide practitioners with the necessary information and guidance to test, specify, and use lithium compounds in new concrete construction, as well as in repair and service life extension applications. This report will be of interest to engineers, contractors, and others involved in the design and specification of new concrete, as well as those involved in mitigation of the damaging effects of alkali-silica reaction in existing concrete structures. Gary L. Henderson, P.E. Director, Office of Infrastructure Research and Development Notice This document is disseminated under the sponsorship of the U.S. Department of Transportation in the interest of information exchange. The U.S. Government assumes no liability for its contents or use thereof. This report does not constitute a standard, specification, or regulation. The U.S. Government does not endorse products or manufacturers. Trade or manufacturers’ names appear herein only because they are considered essential to the objective of this manual. Quality Assurance Statement The Federal Highway Administration (FHWA) provides high-quality information to serve Government, industry, and the public in a manner that promotes public understanding. -
Self-Diffusion in Molten Lithium Nitrate C
Self-Diffusion in Molten Lithium Nitrate C. Herdlicka, J. Richter, and M. D. Zeidler Institut für Physikalische Chemie der Technischen Hochschule Aachen, Aachen, FRG Z. Naturforsch. 47a, 1047-1050 (1992); received July 16, 1992 7 + Self-diffusion coefficients of Li ions have been measured in molten LiN03 with several com- positions of 6Li+ and 7Li+ over a temperature range from 537 to 615 K. The NMR spin-echo method with pulsed field gradients was applied. It was found that the self-diffusion coefficient depends on the isotopic composition and shows a maximum at equimolar ratio. At temperatures above 600 K this behaviour disappears. Key words: Molten salts, Self-diffusion, Isotope effect, NMR spin-echo. Introduction molecular dynamics simulations on LiNOa are not available in which the mass of the ions was varied. It is evident from spectroscopic X-ray [1-3] and Recently, a structural study by neutron diffraction neutron diffraction [4-6] measurements that molten measurements has been carried out on LiN03 melts lithium nitrate shows a special behaviour among the by changing the isotopic composition of either the Li alkali metal nitrates. This is mainly due to the smaller or the N nucleus [11]; the intramolecular structure of size and mass of the Li+-ion, its high polarizing power NOJ has been determined and a local structure of and the stronger cation-anion interactions as com- four nitrate ions around Li+ was deduced. pared with other alkali cations. To improve our un- The self-diffusion coefficients measured by isotopic derstanding of molten salt dynamics the transport tracer techniques refer to the mutual motion of a properties of molten LiNOa are of particular interest, tracer in an unmarked diffusion medium and may be and we deal with self-diffusion in this paper. -
High Themal Energy Storage Density Molten Salts for Parabolic
HIGH THEMAL ENERGY STORAGE DENSITY MOLTEN SALTS FOR PARABOLIC TROUGH SOLAR POWER GENERATION by TAO WANG RAMANA G. REDDY, COMMITTEE CHAIR NITIN CHOPRA YANG-KI HONG A THESIS Submitted in partial fulfillment of the requirements for the degree of Master of Science in the Department of Metallurgical and Materials Engineering in the Graduate School of The University of Alabama TUSCALOOSA, ALABAMA 2011 Copyright Tao Wang 2011 ALL RIGHTS RESERVED ABSTRACT New alkali nitrate-nitrite systems were developed by using thermodynamic modeling and the eutectic points were predicted based on the change of Gibbs energy of fusion. Those systems with melting point lower than 130oC were selected for further analysis. The new compounds were synthesized and the melting point and heat capacity were determined using Differential Scanning Calorimetry (DSC). The experimentally determined melting points agree well with the predicted results of modeling. It was found that the lithium nitrate amount and heating rate have significant effects on the melting point value and the endothermic peaks. Heat capacity data as a function of temperature are fit to polynomial equation and thermodynamic properties like enthalpies, entropies and Gibbs energies of the systems as function of temperature are subsequently induced. The densities for the selected systems were experimentally determined and found in a very close range due to the similar composition. In liquid state, the density values decrease linearly as temperature increases with small slope. Moreover, addition of lithium nitrate generally decreases the density. On the basis of density, heat capacity and the melting point, thermal energy storage was calculated. Among all the new molten salt systems, LiNO3-NaNO3- KNO3-Mg(NO3)2-MgKN quinary system presents the largest thermal energy storage density as well as the gravimetric density values. -
Resorption Heat Transformers Operating with the Ammonia- Lithium Nitrate Mixture
Resorption Heat Transformers Operating with the Ammonia- Lithium Nitrate Mixture J. A. Hernández-Magallanesa, W. Riveraa, A. Coronasb aInstituto de Energías Renovables, Universidad Nacional Autónoma de México (UNAM), 62580 Temixco, Morelos, México. bUniversitat Rovira i Virgili (URV), Av. Paisos Catalans, 26; 43007, Tarragona, Spain. Abstract Absorption heat transformers (AHT) are interesting devices for recovering and upgrading thermal energy which consume very low amount of primary energy. To date, H2O-LiBr is the most common working pair used in absorption heat transformers. However, H2O-LiB mixture has several well-known drawbacks. On the other hand, heat transformers operating with NH3-H2O are not so common because they should operate at very high pressures. The use of a resorption circuit in heat transformers is an interesting alternative to reduce the high pressure level. A resorption heat transformer (RHT) is very similar to an absorption heat transformer, where the evaporator-condenser circuit is replaced by a second desorber-absorber circuit. In the literature it has been reported very few studies about resorption heat transformers operating with the NH3-H2O working pair. In this paper, the modeling and thermodynamic analysis of a single stage absorption heat transformer (AHT) and a single stage resorption heat transformer (RHT) operating with the NH3-LiNO3 mixture is presented. The systems using the NH3-LiNO3 do not require rectification process; therefore the systems using this mixture are simpler than those operating with NH3-H2O. In the present study the coefficients of performance, the exergetic efficiencies and the gross temperature lifts are analyzed as function of the main operating system temperatures. -
Provisional Peer Reviewed Toxicity Values for Lithium (Casrn 7439-93-2)
EPA/690/R-08/016F l Final 6-12-2008 Provisional Peer Reviewed Toxicity Values for Lithium (CASRN 7439-93-2) Superfund Health Risk Technical Support Center National Center for Environmental Assessment Office of Research and Development U.S. Environmental Protection Agency Cincinnati, OH 45268 Acronyms and Abbreviations bw body weight cc cubic centimeters CD Caesarean Delivered CERCLA Comprehensive Environmental Response, Compensation and Liability Act of 1980 CNS central nervous system cu.m cubic meter DWEL Drinking Water Equivalent Level FEL frank-effect level FIFRA Federal Insecticide, Fungicide, and Rodenticide Act g grams GI gastrointestinal HEC human equivalent concentration Hgb hemoglobin i.m. intramuscular i.p. intraperitoneal IRIS Integrated Risk Information System IUR inhalation unit risk i.v. intravenous kg kilogram L liter LEL lowest-effect level LOAEL lowest-observed-adverse-effect level LOAEL(ADJ) LOAEL adjusted to continuous exposure duration LOAEL(HEC) LOAEL adjusted for dosimetric differences across species to a human m meter MCL maximum contaminant level MCLG maximum contaminant level goal MF modifying factor mg milligram mg/kg milligrams per kilogram mg/L milligrams per liter MRL minimal risk level MTD maximum tolerated dose MTL median threshold limit NAAQS National Ambient Air Quality Standards NOAEL no-observed-adverse-effect level NOAEL(ADJ) NOAEL adjusted to continuous exposure duration NOAEL(HEC) NOAEL adjusted for dosimetric differences across species to a human NOEL no-observed-effect level OSF oral slope factor -
Experimental and Theoretical Study of Solubility of New Absorbents in Natural Refrigerants
EXPERIMENTAL AND THEORETICAL STUDY OF SOLUBILITY OF NEW ABSORBENTS IN NATURAL REFRIGERANTS. Javier Mesones Mora Dipòsit Legal: T 1549-2014 ADVERTIMENT. L'accés als continguts d'aquesta tesi doctoral i la seva utilització ha de respectar els drets de la persona autora. Pot ser utilitzada per a consulta o estudi personal, així com en activitats o materials d'investigació i docència en els termes establerts a l'art. 32 del Text Refós de la Llei de Propietat Intel·lectual (RDL 1/1996). Per altres utilitzacions es requereix l'autorització prèvia i expressa de la persona autora. En qualsevol cas, en la utilització dels seus continguts caldrà indicar de forma clara el nom i cognoms de la persona autora i el títol de la tesi doctoral. No s'autoritza la seva reproducció o altres formes d'explotació efectuades amb finalitats de lucre ni la seva comunicació pública des d'un lloc aliè al servei TDX. Tampoc s'autoritza la presentació del seu contingut en una finestra o marc aliè a TDX (framing). Aquesta reserva de drets afecta tant als continguts de la tesi com als seus resums i índexs. ADVERTENCIA. El acceso a los contenidos de esta tesis doctoral y su utilización debe respetar los derechos de la persona autora. Puede ser utilizada para consulta o estudio personal, así como en actividades o materiales de investigación y docencia en los términos establecidos en el art. 32 del Texto Refundido de la Ley de Propiedad Intelectual (RDL 1/1996). Para otros usos se requiere la autorización previa y expresa de la persona autora. -
HR2-697 Lithium Nitrate
Safety Data Sheet According to Regulation (EC) No 1907/2006 Revision Date: 03/23/2020 Version: 1.4 Date Printed: GENERIC EU MSDS - NO COUNTRY SPECIFIC DATA - NO OEL DATA SECTION 1: Identification of the substance/mixture and of the company/undertaking 1.1 Product identifiers Product Name : 8.0 M Lithium nitrate Product Number : HR2-697 REACH No. : A registration number is not available for this substance as the substance or its uses are exempted from registration, the annual tonnage does not require a registration or the registration is envisaged for a later registration deadline. CAS Number : 7790-69-4 1.2 Relevant identified uses of the substance or mixture and uses advised against Identified uses : Laboratory chemicals, Manufacture of substances. 1.3 Details of the supplier of the Safety Data Sheet Company : Hampton Research 34 Journey Aliso Viejo, CA 92656-3317 United States Telephone : 949 425 1321 Telephone technical support is available 8:00 a.m. to 4:30 p.m. USA Pacific Standard Time. Fax : 949 425 1611 Fax Technical Support is available 24 hours a day. e-mail : [email protected] e-mail Technical Support is available 24 hours a day. 1.4 Emergency telephone number Emergency phone : 949 425 1321 For CHEMTREC Assistance : 800 424 9300 For CHEMTREC Assistance : 703 527 3887 (International) SECTION 2: Hazards Identification 2.1 Classification of the substance or mixture Classification according to Regulation (EC) No 1272/2008 Oxidizing solids (Category 3), H272 For the full text of the H-Statements mentioned in this Section, see Section 16. Classification according to EU Directives 67/548/EEC or 1999/45/EC O Oxidising R 8 For the full text of the R-phrases mentioned in this Section, see Section 16.