Gradient-Index-Based Frequency-Coded Retroreflective Lenses for Mm-Wave Indoor Localization
Total Page:16
File Type:pdf, Size:1020Kb
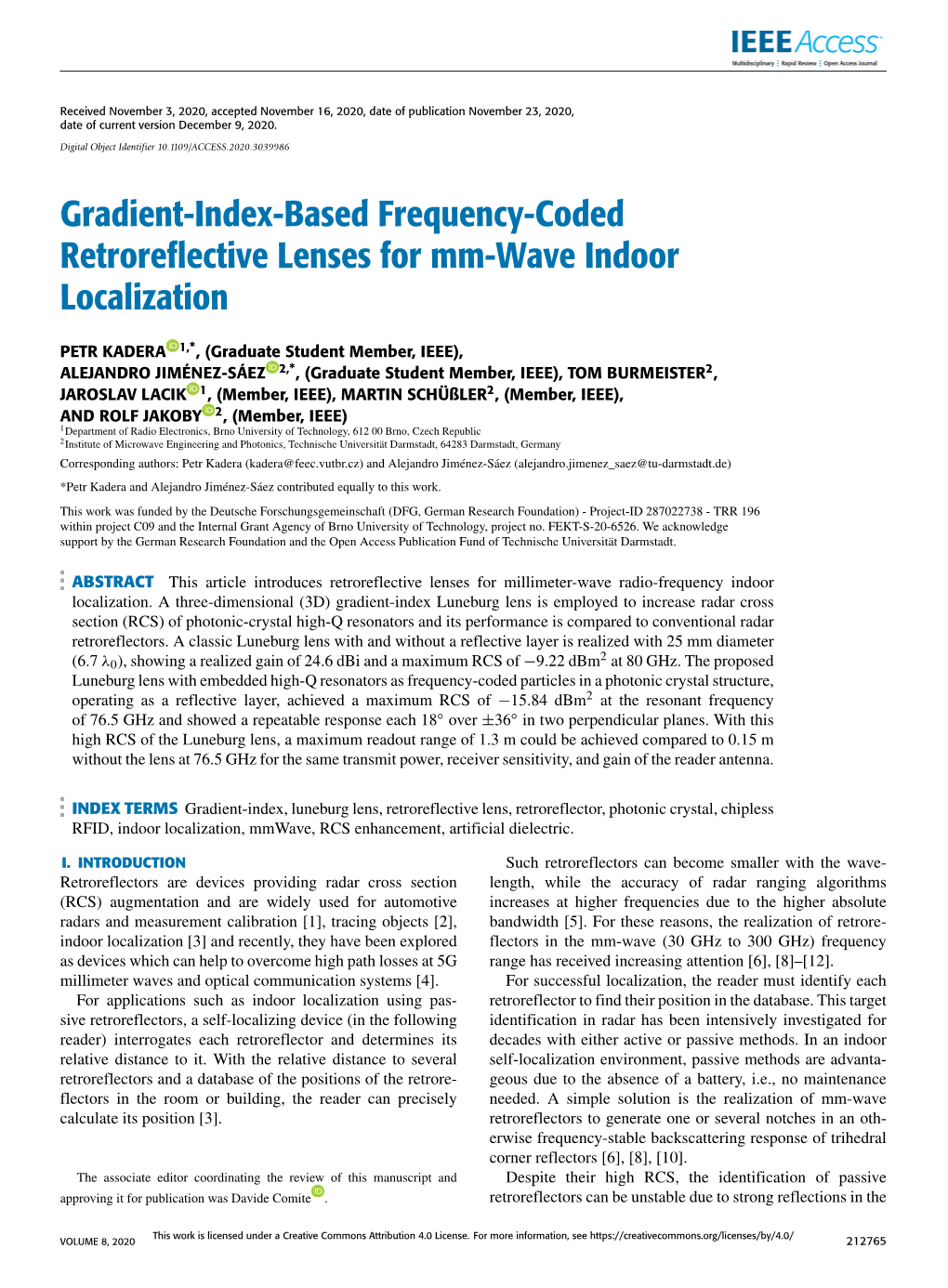
Load more
Recommended publications
-
Download Full Article
Progress In Electromagnetics Research, PIER 72, 325–337, 2007 RADAR CROSS-SECTION STUDIES OF SPHERICAL LENS REFLECTORS S. S. Vinogradov CSIRO ICT Centre PO Box 76, Epping, NSW, 1710, Australia P. D. Smith Department of Mathematics Division of ICS, Macquarie University NSW 2109, Australia J. S. Kot and N. Nikolic CSIRO ICT Centre PO Box 76, Epping, NSW, 1710, Australia Abstract—The reflectivity of a Spherical Lens Reflector is investi- gated. The scattering of an electromagnetic plane wave by a Spherical Lens Reflector is treated as a classical boundary value problem for Maxwell’s equations. No restrictions are imposed on the electrical size of reflectors and the angular size of the metallic spherical cap. The competitiveness of the Spherical Lens Reflector against the Luneberg Lens Reflector is demonstrated. It has been found that Spherical Lens Reflectors with relative dielectric constant in the range 3.4 ≤ εr ≤ 3.7 possess better spectral performance than 3- or 5-layer Luneberg Lens Reflectors in a wide frequency range. 1. INTRODUCTION The Spherical Lens (SL) is a homogeneous dielectric sphere [1] which, for all dielectric constants in the range 1 ≤ εr ≤ 4, focuses paraxial rays to a point zGO outside the sphere. The distance from the centre of the lens to zGO is f, the paraxial focal length, which may be determined 326 Vinogradov et al. by Geometrical Optics, and is given in normalized form by √ f ε = √ r (1) r1 2 · ( εr − 1) where r1 is the radius of the SL. At microwave frequencies the more popular choice for the design of efficient reflectors is a stepped-index Luneburg Lens (LL) with attached metallic spherical cap [2–6]. -
State-Of-The-Art of Metamaterials: Characterization, Realization and Applications
Technological University Dublin ARROW@TU Dublin Articles Antenna & High Frequency Research Centre 2014-7 State-of-the-Art of Metamaterials: Characterization, Realization and Applications Giuseppe Ruvio Technological University Dublin, [email protected] Giovanni Leone Second University of Naples, [email protected] Follow this and additional works at: https://arrow.tudublin.ie/ahfrcart Part of the Electromagnetics and Photonics Commons Recommended Citation G. Ruvio & G. Leone,(2014) State-of-the-art of Metamaterials: Characterization, Realization and Applications, Studies in Engineering and Technology, vol. 1, issue 2. doi:10.11114/set.v1i2.456 This Article is brought to you for free and open access by the Antenna & High Frequency Research Centre at ARROW@TU Dublin. It has been accepted for inclusion in Articles by an authorized administrator of ARROW@TU Dublin. For more information, please contact [email protected], [email protected]. This work is licensed under a Creative Commons Attribution-Noncommercial-Share Alike 4.0 License Redfame Publish ing State-of-the-art of metamaterials: characterization, realization and applications Giuseppe Ruvio 1, 2 & Giovanni Leone 1 1Seconda Università di Napoli, Dipartimento di Ingegneria Industriale e dell’Informazione, Via Roma 29, 81031 Aversa (CE), Italy 2Dublin Institute of Technology, Antenna & High Frequency Research Centre, Kevin Street, Dublin 8, Ireland Abstract Metamaterials is a large family of microwave structures that produces interesting ε and µ conditions with huge implications for numerous electromagnetic applications. Following a description of modern techniques to realize epsilon-negative, mu-negative and double-negative metamaterials, this paper explores recent literature on the use of metamaterials in hot research areas such as metamaterial-inspired microwave components, antenna applications and imaging. -
Foam Based Luneburg Lens Antenna at 60 Ghz
Progress In Electromagnetics Research Letters, Vol. 44, 1{7, 2014 Foam Based Luneburg Lens Antenna at 60 GHz Jonathan Bor1, *, Olivier Lafond1, Herve Merlet2, Philippe Le Bars2, and Mohamed Himdi1 Abstract|An innovative technological process is investigated to easily manufacture inhomogeneous Luneburg lenses. A unique foam material is drilled and pressed to achieve the di®erent dielectric constant needed to follow the index law inside the lens. The performance of such 60 GHz antenna is described and the antenna prototype is measured in terms of gain and radiation patterns. The results show a good e±ciency (60% with a directivity of 18{19 dBi) and demonstrate the feasibility of this kind of Luneburg lens, through the use of a simple technological process. The lens with a diameter of 56 mm and a thickness of 3 mm operates in the 57{66 GHz bandwidth. The magnitude of S11 parameter is under ¡10 dB in the whole bandwidth and an half-power beamwidth of 5± and 50± in H-plane and E-plane respectively is reached. 1. INTRODUCTION Millimeter-wave communication systems in the unlicensed 57{66 GHz bandwidth are dedicated for indoor and short range digital high rate transmission applications [1]. In indoor conditions, the link between transmitter and receiver can be shadowed because of human body interposition for example. Therefore, beam-scanning antennas are required to carry out high bite rate communications to another receptor. Luneburg lens have been chosen for its low loss, low retro-di®usion and in¯nity of focus points for beam scanning and beam shaping capabilities. -
The Talbot Effect, Lüneburg Lenses & Metamaterials
Optical Switch on a Chip: The Talbot Effect, Lüneburg Lenses & Metamaterials Hamdam Nikkhah Thesis submitted to the Faculty of Graduate and Postdoctoral Studies in partial fulfillment of the requirements for the degree of Master of Science Systems Science Program School of Electrical Engineering and Computer Science University of Ottawa Ottawa, Canada © Hamdam Nikkhah, Ottawa, Canada, 2013 Abstract The goal of the research reported in this thesis is to establish the feasibility of a novel optical architecture for an optical route & select circuit switch suitable for implementation as a photonic integrated circuit. The proposed architecture combines Optical Phased Array (OPA) switch elements implemented as multimode interference coupler based Generalised Mach- Zehnder Interferometers (GMZI) with a planar Lüneburg lens-based optical transpose interconnection network implemented using graded metamaterial waveguide slabs. The proposed switch is transparent to signal format and, in principle, can have zero excess insertion loss and scale to large port counts. These switches will enable the low-energy consumption high capacity communications network infrastructure needed to provide environmentally-friendly broadband access to all. The thesis first explains the importance of switch structures in optical communications networks and the difficulties of scaling to a large number of switch ports. The thesis then introduces the Talbot effect, i.e. the self-imaging of periodic field distributions in free space. It elaborates on a new approach to finding the phase relations between pairs of Talbot image planes at carefully selected positions. The free space Talbot effect is mapped to the waveguide Talbot effect which is fundamental to the operation of multimode interference couplers (MMI). -
A Luneburg Lens for the Terahertz Region
Journal of Infrared, Millimeter, and Terahertz Waves https://doi.org/10.1007/s10762-019-00635-8 A Luneburg Lens for the Terahertz Region Yasith Amarasinghe1 & Daniel M. Mittleman1 & Rajind Mendis1,2 Received: 21 August 2019 /Accepted: 14 October 2019/ # Springer Science+Business Media, LLC, part of Springer Nature 2019 Abstract We experimentally demonstrate a two-dimensional Luneburg lens for the THz region using a waveguide-based artificial-dielectric medium. The substrate material of the lens is Teflon with the top and bottom surfaces coated with silver to form a quasi-parallel-plate waveguide. The top surface of the device has a curved conical profile and the bottom surface is flat. The lens can focus an approximately 2-cm diameter input beam at a frequency of 0.162 THz to a spot size of 3.4 mm (less than 2λ) at the diametrically opposite edge of the device. Keywords Gradient-index lenses . Artificial dielectrics . Spectroscopy, terahertz . Luneburg lens A gradient-index (GRIN) lens is an example of an optical device whose focusing effect is realized by a spatial variation of the refractive index of the constituent material [1]. The design and fabrication of devices like GRIN lenses using naturally occurring dielectrics is an ongoing challenge [2]. In particular, it is difficult to realize a continuously varying refractive index, and in some cases, the required high index gradients. Some of the fabrication methods which have been used to approximate a gradient index are the onion-shell technique [3], the tapered-hole approach [4],theslicetechnique[5], the sub-wavelength microstructuring method [6], and 3D printing methods [7, 8]. -
Broadband Wide-Angle Terahertz Antenna Based on the Application Of
www.nature.com/scientificreports OPEN Broadband wide‑angle terahertz antenna based on the application of transformation optics to a Luneburg lens Yasith Amarasinghe1, Rajind Mendis2, Rabi Shrestha1, Hichem Guerboukha1, Jochen Taiber3, Martin Koch3 & Daniel M. Mittleman1* The design of antennas for terahertz systems remains a signifcant challenge. These antennas must provide very high gain to overcome signifcant free‑space path loss, which limits their ability to broadcast or receive a beam over a wide angular range. To circumvent this limitation, here we describe a new device concept, based on the application of quasi‑conformal transformation optics to the traditional Luneburg lens. This device ofers the possibility for wide‑angle beam steering and beam reception over a broad bandwidth, scalable to any frequency band in the THz range. It has long been recognized that high-frequency communications links, operating above 100 GHz, will require high-gain antennas to overcome the large free-space path loss 1,2. As a result, most researchers envision that terahertz wireless networks will employ highly directional, pencil-like beams, rather than omni-directional broadcasts that are commonly used in existing wireless systems. Tese terahertz systems will therefore require fast and agile beam steering. Of course, this could be accomplished using integrated phased arrays3. However, the implementation of integrated beam steering capabilities is still challenging in the terahertz range due mainly to the lack of practical phase shifers4. A variety of alternative solutions have been proposed, including mechanical systems5,6, liquid crystal devices7, and metasurfaces8–10. Another possibility is enabled by the concepts of transformation optics 11,12, which can be applied to well- known spherically symmetric lens designs to produce valuable new capabilities13. -
Ka-Band 2D Luneburg Lens Design with Glide-Symmetric Metasurface
DEGREE PROJECT IN ELECTRICAL ENGINEERING, SECOND CYCLE, 30 CREDITS STOCKHOLM, SWEDEN 2017 Ka-band 2D Luneburg Lens Design with Glide-symmetric Metasurface JINGWEI MIAO KTH ROYAL INSTITUTE OF TECHNOLOGY SCHOOL OF ELECTRICAL ENGINEERING TRITA 2017:115 ISSN 1653-5146 www.kth.se Ka-band 2D Luneburg Lens Design with Glide-symmetric Metasurface Jingwei Miao Supervisors: Astrid Algaba Brazalez, Lars Manholm, Martin Johansson N (Ericsson) Fatemeh Ghasemifard (KTH) Examiner: Oscar Quevedo-Teruel (KTH) A thesis submitted for the degree of Master of Science July 2017 Abstract A Luneburg lens is a beam former that has two focal points where one is at the surface and the other lies at infinity. It is a cheap passive steerable antenna at high frequencies. In this thesis, a 2D flat-profile Luneburg lens with all-metal structure is designed for Ka band. Commercial soft- ware CST Microwave Studio Suite and Ansys Electronic Desktop (HFSS) are used for simulations. The lens is composed of two glide-symmetric metasurface layers with a small gap in between. The high order symmetry, glide symmetry, could provide ultra wide band property for the lens. Each layer contains many unit cells. Different unit cells are tested in this thesis to find the best solu- tion taking into account both electromagnetic properties and the easiness of manufacturing. A flare is designed to achieve better matching between the air gap of the lens and free space. A self-designed waveguide feeding is also used, including a transition from coaxial cable to TE10 mode of rectangular waveguide at the focus of the lens. The prototype will be built in the future and measurements will be done to compare with simulation results in this thesis. -
Modeling of a Stepped Luneburg Lens for All-Sky Surveys
Modeling of a stepped Luneburg lens for all-sky surveys Mason Carneya and Matthew A. Kenworthya aLeiden Observatory, Leiden University, P.O. Box 9513, 2300 RA Leiden, The Netherlands ABSTRACT We investigate the scattered light properties of a Luneburg lens approximated as a series of concentric shells with discrete refractive indices. The stepped Luneburg lens has been previously modeled at microwave wavelengths with full solutions for the electromagnetic field equations when the lens is of comparable size to the wavelength. We investigate the properties of a Luneburg lens at optical wavelengths using a geometric ray tracing technique. We develop a stack-based ray tracing algorithm with the python programming language that tracks all reflected and refracted rays generated at each optical interface. The code shows that a Luneburg lens with 40 steps and a refractive index power-law exponent of 0.55 will produce images of nearly all naked eye (<6) magnitude stars with an enclosed energy of 50% at a spatial resolution of 3.2 degrees. We find 72 cases of blended stars where a star with magnitude <6 falls within 3 degrees of angular separation from a star with magnitude < 1. The optical stepped Luneburg lens has promising applications for low-cost, continuous all-sky monitoring to obtain transit light curves of bright, nearby stars. Keywords: Luneberg lens, optics, ray tracing 1. INTRODUCTION A Luneburg lens is a spherically symmetric optic with a variable index of refraction n that is a function of the radius r such that n = n(r). The equations that govern the relationship between n and r can give the lens the ability to form perfect geometrical images of two concentric spheres onto one another. -
Compact Acoustic Retroreflector Based on a Mirrored Luneburg
Compact Acoustic Retroreflector Based on A Mirrored Luneburg Lens Yangyang Fu1,2,3,*, Junfei Li1, *, Yangbo Xie1, *, Chen Shen1, Yadong Xu3, Huanyang Chen2,† and Steven A. Cummer1,‡ 1. Department of Electrical and Computer Engineering, Duke University, Durham, North Carolina 27708, USA 2. Institute of Electromagnetics and Acoustics and Department of Electronic Science, Xiamen University, Xiamen 361005, China. 3. College of Physics, Optoelectronics and Energy, Soochow University, No.1 Shizi Street, Suzhou 215006, China. We propose and demonstrate a compact acoustic retroreflector that reroutes probing signals back towards the source with minimal scattering. Gradient refractive index (GRIN) acoustic metamaterials, based on Archimedean spiral structures, are designed to fulfill the required refractive index profile. The experiments show that the compact acoustic retroreflector, whose radius is only approximately one wavelength, works in an incident angular range up to 120 degrees over a relatively broad bandwidth of about 27% of the central frequency. Such compact acoustic retroreflectors can be potentially applied in pulse-echo based acoustic detection and communication, such as unmanned aerial vehicle (UAV) sonar systems, robotic ranging detectors, ultrasonic imaging systems. I. INTRODUCTION A retroreflector is a volumetric or surface structure that redirects the incident wave back towards the source, along the direction parallel but opposite to the direction of incidence. By rerouting the incident probing signal back towards the source or the transceiver, less signal is wasted in the uncontrolled scattering process and higher signal-to-noise (SNR) ratio of the sensing signal is obtained. In optics, conventional optical retroreflectors, realized with corner reflector or cat’s eye configurations, can work within limited incident angles. -
Dielectric Lens Antennas
Dielectric Lens Antennas Carlos A. Fernandes, Instituto de Telecomunicações, Instituto Superior Técnico, Universidade de Lisboa, Lisboa, Portugal Eduardo B. Lima, Instituto de Telecomunicações, Instituto Superior Técnico, Universidade de Lisboa, Lisboa, Portugal Jorge R. Costa, Instituto de Telecomunicações, Instituto Universitário de Lisboa (ISCTE- IUL), Lisboa, Portugal ABSTRACT Dielectric lens antennas are attracting a renewed interest for millimetre- and sub- millimetre wave applications where they become compact, especially for configurations with integrated feeds usually referred as integrated lens antennas. Lenses are very flexible and simple to design and fabricate, being a reliable alternative at these frequencies to reflector antennas. Lens target output can range from a simple collimated beam (increasing the feed directivity) to more complex multi-objective specifications. This chapter presents a review of different types of dielectric lens antennas and lens design methods. Representative lens antenna design examples are described in detail, with emphasis on homogeneous integrated lenses. A review of the different lens analysis methods is performed, followed by the discussion of relevant lens antenna implementation issues like feeding options, dielectric material characteristics, fabrication methods and a few dedicated measurement techniques. The chapter ends with a detailed presentation of some recent application examples involving dielectric lens antennas. KEYWORDS Lens Antennas, Geometric Optics, Physical Optics, Lens Feeds, Dielectric Materials, Lens Manufacturing, Lens Profile Design, Optimization. INTRODUCTION The use of a dielectric lens as part of an antenna is almost as old as the demonstration of electromagnetic waves by Hertz. In fact, in 1888 Oliver Lodge used a dielectric lens in his experiments at 1 m wavelength (Lodge and Howard 1888). -
Three-Dimensional Gradient-Index Materials and Their Applications In
IEEE TRANSACTIONS ON ANTENNAS AND PROPAGATION, VOL. 61, NO. 5, MAY 2013 2561 Three-Dimensional Gradient-Index Materials and Their Applications in Microwave Lens Antennas Hui Feng Ma, Ben Geng Cai, Teng Xiang Zhang, Yan Yang, Wei Xiang Jiang, and Tie Jun Cui, Senior Member, IEEE Abstract—In this paper, we introduce a method to realize 3-D of the lens. Half-spherical Luneburg lens and half fisheye lens inhomogeneous and nearly isotropic gradient-index materials in have also important applications as high-directive microwave the microwave regime. The unit cells of such gradient-index mate- antennas [5]–[8]. Traditionally, the Luneburg and fisheye lenses rials are drilled-hole dielectric structures, which can be easily fab- ricated by using the traditional printed circuit boards. To demon- have been produced by a series of concentric inhomogeneous strate the feasibility of the proposed method, we design and realize dielectric shells, whose electric permittivity is varied discretely two functional devices in the microwave frequencies—a half-spher- from 1 to 4 [5]–[10]. However, the fabrication of such con- ical Luneburg lens and a half Maxwell fisheye lens—based on 3-D centric dielectric shells is quite complicated and there exists gradient-index materials. The measurement results show that both impedance mismatch among dielectric shells, which will de- devices have very good performance, which have good agreements to the numerical simulations, illustrating the great application po- grade the performance. There have been some interesting works tentials of the 3-D gradient-index materials. for designing the Luneburg lenses byusingartificial electro- magnetic structures [11]–[14]: the refractive index of the lens is Index Terms—Gradient-index materials, Luneburg lens, Maxwell fisheye lens, three dimensions. -
Analytical, Numerical, and Experimental Investigation of a Luneburg Lens System for Directional Cloaking
Analytical, numerical, and experimental investigation of a Luneburg lens system for directional cloaking C. Babayigit,˘ 1,* Aydın S. Evren,2 E. Bor,1,2 H. Kurt,1 and M. Turduev2 1Department of Electrical and Electronics Engineering, TOBB University of Economics and Technology, Ankara 06560, Turkey 2Department of Electrical and Electronics Engineering, TED University, Ankara 06420, Turkey In this study, the design of a directional cloaking based on the Luneburg lens system is proposed and its operating principle is experimentally verified. The cloaking concept is analytically investigated via geometrical optics and numerically realized with the help of the finite-difference time-domain method. In order to benefit from its unique focusing and/or collimating characteristics of light, the Luneburg lens is used. We show that by the proper combination of Luneburg lenses in an array form, incident light bypasses the region between junctions of the lenses, i.e., the “dark zone.” Hence, direct interaction of an object with propagating light is prevented if one places the object to be cloaked inside that dark zone. This effect is used for hiding an object which is made of a perfectly electric conductor material. In order to design an implementable cloaking device, the Luneburg lens is discretized into a photonic crystal structure having gradually varying air cylindrical holes in a dielectric material by using Maxwell Garnett effective medium approximations. Experimental verifications of the designed cloaking structure are performed at microwave frequencies of around 8 GHz. The proposed structure is fabricated by three-dimensional printing of dielectric polylactide material and a brass metallic alloy is utilized in place of the perfectly electric conductor material in microwave experiments.