Lorna Wilkie Phd Thesis
Total Page:16
File Type:pdf, Size:1020Kb
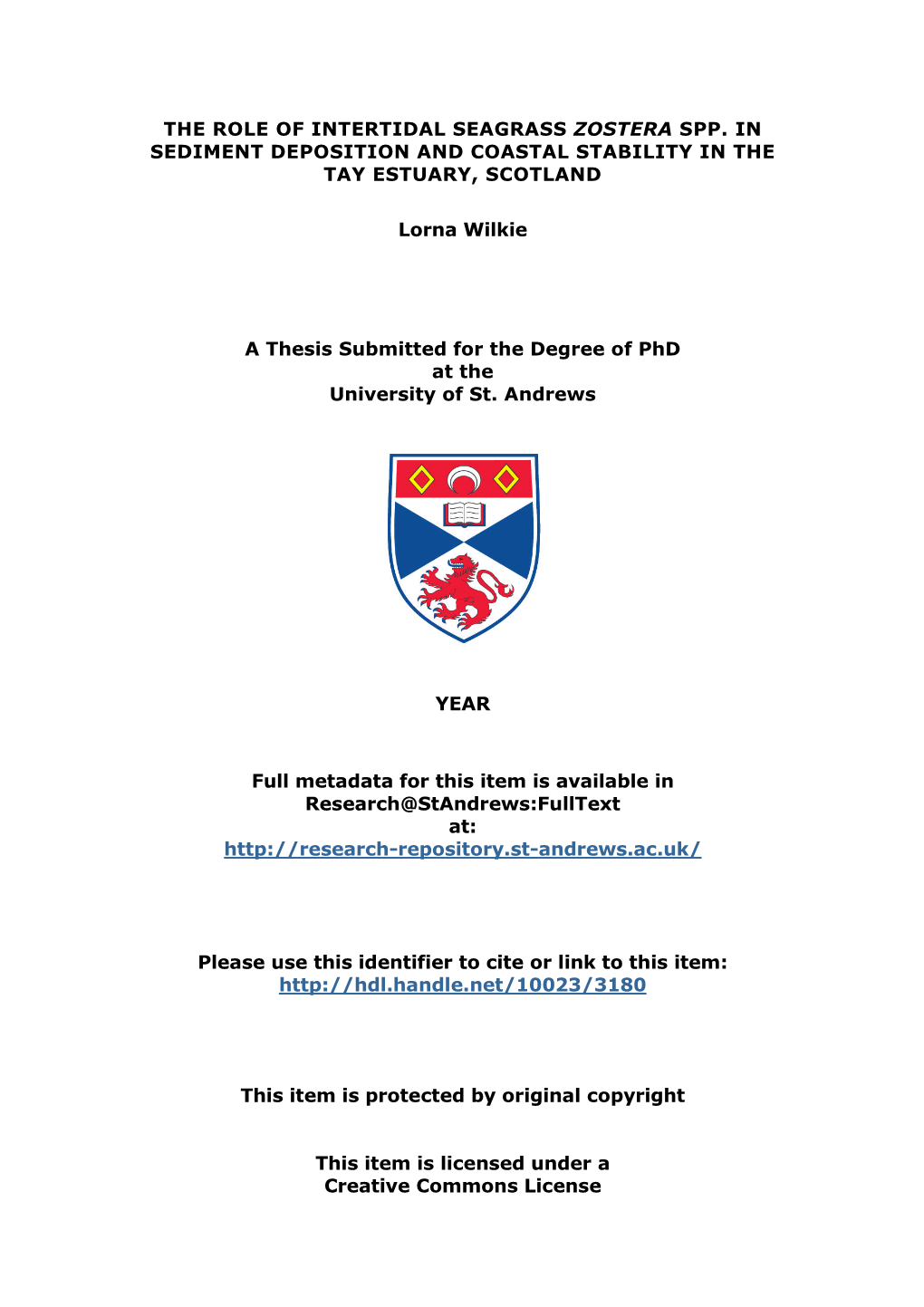
Load more
Recommended publications
-
MD17 Bus Time Schedule & Line Route
MD17 bus time schedule & line map MD17 St Andrews, Madras College - Tayport, Queen View In Website Mode Street The MD17 bus line St Andrews, Madras College - Tayport, Queen Street has one route. For regular weekdays, their operation hours are: (1) Tayport: 4:07 PM Use the Moovit App to ƒnd the closest MD17 bus station near you and ƒnd out when is the next MD17 bus arriving. Direction: Tayport MD17 bus Time Schedule 59 stops Tayport Route Timetable: VIEW LINE SCHEDULE Sunday Not Operational Monday 4:07 PM New Madras College, St Andrews Tuesday 5:07 PM Strathtyrum Golf Course, St Andrews Wednesday 4:07 PM Easter Kincaple Farm, Kincaple Thursday 5:07 PM Edenside, Kincaple Friday 2:37 PM Guardbridge Hotel, Guardbridge Saturday Not Operational Mills Building, Guardbridge Ashgrove Buildings, Guardbridge MD17 bus Info Innerbridge Street, Guardbridge Direction: Tayport Stops: 59 Innerbridge Street, Scotland Trip Duration: 70 min Line Summary: New Madras College, St Andrews, Toll Road, Guardbridge Strathtyrum Golf Course, St Andrews, Easter Kincaple Farm, Kincaple, Edenside, Kincaple, Station Road, Leuchars Guardbridge Hotel, Guardbridge, Mills Building, Guardbridge, Ashgrove Buildings, Guardbridge, St Bunyan's Place, Leuchars Innerbridge Street, Guardbridge, Toll Road, Guardbridge, Station Road, Leuchars, St Bunyan's Fern Place, Leuchars Place, Leuchars, Fern Place, Leuchars, Cemetery, A919, Leuchars Leuchars, Castle Farm Road End, Leuchars, Dundee Road, St Michaels, Inn, Pickletillem, National Golf Cemetery, Leuchars Centre, Drumoig, Forgan -
Derek and Moira Lawson
For unto you is born this day in the city of David a Saviour, which is Christ the Lord. Luke 2:11 The Quarterly Newsletter of Christ Church, Warwick, Bermuda Derek and Moira Lawson Derek was born in Dundee, Scotland, on 2nd February 1949, the eldest child (of 3) born to Fettes and Lily Lawson. Moira was privileged (her word) to be born in Edinburgh on 17th September 1951, the elder of 2 daughters born to Norman and Margaret Leighton. Both grew up as regular church attenders – Derek at Dundee Parish Church (St Mary’s) and Moira at St. Aidan’s, Stenhouse, in Edinburgh – and were both active in their congregations. While many teenagers in the 60s drifted away from the church, Derek’s con- tact was, if anything, strengthened through his attending the Youth Camps on Iona (a small is- land off the west coast of Scotland, and the centre of the Iona Community) over three summers. Indeed, looking back, that’s probably where the idea of involvement in ministry had its origin, and, even today, Derek remains an Associate Member of the Iona Community. However, as his father was a solicitor and Derek worked in the family firm during summer holi- days (as a kind of glorified office messenger!), his career path was set and so in 1966 he started his law course at Queen’s College, Dundee (part of the University of St Andrews). He completed his LL.B. degree in 1969 and was one of the first graduates of the University of Dundee – Queen’s College having by then gained the status of an independent university. -
54, Riverside Road , Wormit, Newport on Tay, Dd6
HOME REPORT 54 RIVERSIDE ROAD WORMIT NEWPORT ON TAY DD6 8LJ ENERGY PERFORMANCE CERTIFICATE YouEnergy can use this Performance document to: Certificate (EPC) Scotland Dwellings 54 RIVERSIDE ROAD, WORMIT, NEWPORT ON TAY, DD6 8LJ Dwelling type: Semi-detached house Reference number: 4800-1532-0622-3124-1803 Date of assessment: 27 August 2020 Type of assessment: RdSAP, existing dwelling Date of certificate: 31 August 2020 Approved Organisation: Elmhurst Total floor area: 118 m2 Main heating and fuel: Boiler and radiators, mains Primary Energy Indicator: 301 kWh/m2/year gas You can use this document to: • Compare current ratings of properties to see which are more energy efficient and environmentally friendly • Find out how to save energy and money and also reduce CO2 emissions by improving your home Estimated energy costs for your home for 3 years* £3,891 See your recommendations report for more Over 3 years you could save* £1,662 information * based upon the cost of energy for heating, hot water, lighting and ventilation, calculated using standard assumptions Very energy efficient - lower running costs Current Potential Energy Efficiency Rating (92 plus) A This graph shows the current efficiency of your home, (81-91) B 86 taking into account both energy efficiency and fuel costs. The higher this rating, the lower your fuel bills (69-80) C are likely to be. (55-68) D 60 Your current rating is band D (60). The average rating for EPCs in Scotland is band D (61). (39-54 E (21-38) The potential rating shows the effect of undertaking all F of the improvement measures listed within your (1-20) G recommendations report. -
Two Plots, W Est Park R Oad, W Ormit
Two Plots, West Park Road, Wormit DD6 Two Plots for Two New Build Executive Villas Approved planning permission for Detached Villa comprising Two building plots, with river views, for two architect-designed five bed executive detached villas. Planning permission and building warrants in place. Detached Entrance Hall A rarely available self-build opportunity in this quiet, popular residential cul-de-sac on Sitting Room the banks of the River Tay. Open Plan Kitchen Dining Plots sizes as follow: Family Room/Bedroom 5 Plot 1: 612 m sq. Master Bedroom En Suite Shower Room Plot 2: 636 m sq. Dressing Room If built as per the approved plans, each property will comprise three levels with Three Double Bedrooms adaptable accommodation. Family Bathroom Location Cloakroom The attractive village of Wormit hosts a thriving and friendly community. Within walking Utility Room distance of the property is an excellent primary school. The village is a haven for water Sun Terrace and Balcony with views of the River sports enthusiasts who like sailing, water-skiing and fishing. There are also tennis and Tay bowling clubs. Young families are well served with a broad range of children’s activity Plot 1 has store/play room on the ground floor clubs. Nearby Newport-on-Tay offers a modern, sophisticated array of shops and Plot 2 has 5th bedroom on the ground services. Its High Street boasts a new art gallery, cafes, a renowned hair and beauty salon, gift shops and a florist, as well as the usual convenience stores and post office. Double Glazing There is a doctor’s surgery and chemist in the village as well as a library. -
Gauldry G Wormit G Newport G Dundee G Ninewells G Invergowrie
bustimes from 2nd February 2015 page 1 of 4 Gauldry G Wormit G Newport G Dundee G Ninewells G Invergowrie G Kingoodie 77 MONDAYS TO FRIDAYS route number 77 77 77B 77 77B 77 77B 77 77B 77 77B 77 77B 77 77B 77 77B 77 77B 77 Gauldry Main Rd Windmill Rd — — — 0752 — 0857 — 1004 — 1104 — 1204 — 1304 — 1404 — 1504 — 1604 Gauldry Balgove Road — — — M — M — 1007 — 1107 — M — 1307 — M — 1507 — 1607 Balmerino road end — — — 0757 — 0902 — M — M — 1209 — M — 1409 — M — M Wormit opp post offi ce 0640 0705 0735 0805 0835 0910 0940 1015 1040 1115 1140 1217 1240 1315 1340 1417 1440 1515 1540 1615 Newport Kinbrae Park 0645 0711 0741 0812 0841 0916 0946 1021 1046 1121 1146 1223 1246 1321 1346 1423 1446 1521 1546 1621 Newport High Street 0647 0713 0743 0815 0843 0918 0948 1023 1048 1123 1148 1225 1248 1323 1348 1425 1448 1523 1548 1623 Newport Norwood M M 0747 M 0847 M 0952 M 1052 M 1152 M 1252 M 1352 M 1452 M 1552 M Tay Road Bridge south access 0650 0717 0752 0820 0852 0922 0957 1027 1057 1127 1157 1229 1257 1327 1357 1429 1457 1527 1557 1627 Dundee rail station 0655 | 0757 | 0857 | 1002 | 1102 | 1202 | 1302 | 1402 | 1502 | 1602 | Dundee Crichton Street 0657 | 0800 | 0901 | 1005 | 1105 | 1205 | 1305 | 1405 | 1505 | 1605 | Dundee bus station 0701 M 0806 M 0906 M 1009 M 1109 M 1209 M 1309 M 1409 M 1509 M 1609 M Dundee Seagate opp M&S — 0723 — 0828 — 0928 — 1033 — 1133 — 1235 — 1333 — 1435 — 1533 — 1633 Dundee Whitehall Street — 0727 — 0832 — 0932 — 1037 — 1137 — 1239 — 1337 — 1439 — 1537 — 1637 Hawkhill University — 0731 — 0837 — 0936 — 1041 — 1141 — 1243 -
New SNH Firth of Tay/Eden
COMMISSIONED REPORT Commissioned Report No. 007 Broad scale mapping of habitats in the Firth of Tay and Eden Estuary, Scotland (ROAME No. F01AA401D) For further information on this report please contact: Dan Harries Maritime Group Scottish Natural Heritage 2 Anderson Place EDINBURGH EH6 5NP Telephone: 0131–446 2400 E-mail: [email protected] This report should be quoted as: Bates, C. R., Moore, C. G., Malthus, T., Mair, J. M. and Karpouzli, E. (2004). Broad scale mapping of habitats in the Firth of Tay and Eden Estuary, Scotland. Scottish Natural Heritage Commissioned Report No. 007 (ROAME No. F01AA401D). This report, or any part of it, should not be reproduced without the permission of Scottish Natural Heritage. This permission will not be withheld unreasonably. The views expressed by the author(s) of this report should not be taken as the views and policies of Scottish Natural Heritage. © Scottish Natural Heritage 2003. Scottish Natural Heritage Commissioned Report No. 007 (ROAME No. F01AA401D) This report was produced for Scottish Natural Heritage by the Sedimentary Systems Research Unit, University of St Andrews, the School of Life Sciences Heriot-Watt University and the Department of Geography, University of Edinburgh on the understanding that the final data provided can be used only by these parties and SNH. Dr Richard Bates Sedimentary Systems Research Unit School of Geography and Geosciences University of St Andrews St Andrews Dr Colin Moore School of Life Sciences Heriot-Watt University Edinburgh Dr Tim Malthus Department of Geography University of Edinburgh Edinburgh SUPPORTING INFORMATION: Scottish Natural Heritage holds all other non-published data products arising from this mapping project including raw sediment PSA data, video footage, raw acoustic data and GIS products. -
Mute Swan Cygnus Olor (Britain and Ireland Populations) in Britain and Northern Ireland 1960/61 – 2000/01
Mute Swan Cygnus olor (Britain and Ireland populations) in Britain and Northern Ireland 1960/61 – 2000/01 Helen Rowell1 & Chris Spray2 with contributions from Tim Appleton, Richard Averiss, Andrew Bramhall, Anne Brenchley, Allan & Lyndesay Brown, Graham Catley, Helen Chisholm, Jon Coleman, Ilona & Terry Coombs, Colin Corse, Bill Curtis, Ian Enlander, Stephen Foster, Robert Gardiner, Wes Halton, Richard Humpidge, John Leece, Bruce Martin, Eric Meek, Steve Meen, Dave Paynter, Craig Ralston, Jack Sheldon, Darrell Stevens, David Stone, Johne Taylor, Matthew Tickner, Rick Vonk, Sian Whitehead and Bernie Zonfrillo 1 The Wildfowl & Wetlands Trust, Slimbridge, Glos GL2 7BT, UK 2 Chapel View, Hamsterley, Bishop Auckland, Co Durham DL13 3PP, UK Waterbird Review Series © The Wildfowl & Wetlands Trust/Joint Nature Conservation Committee All rights reserved. Apart from any fair dealing for the purpose of private study, research, criticism or review (as permitted under the Copyright Designs and Patents Act 1988), no part of this publication may be reproduced, sorted in a retrieval system or transmitted in any form or by any means, electronic, electrical, chemical, optical, photocopying, recording or otherwise, without prior permission of the copyright holder. ISBN 0 900806 39 7 This publication should be cited as: Rowell, HE & CJ Spray. 2004. The Mute Swan Cygnus olor (Britain and Ireland populations) in Britain and Northern Ireland 1960/61 – 2000/01. Waterbird Review Series, The Wildfowl & Wetlands Trust/Joint Nature Conservation Committee, Slimbridge. -
Dundee Property Magazine
TAYSIDE FEBRUARY 2020 Thinking of moving? Now’s the time To find more properties for sale, visit our new website property.lindsays.co.uk FEBRUARY 2020 Looking to sell in 2020? There are always plenty of unknowns in the There are other reasons to sell with us too: housing market, but there’s one rule that • We know the market. Demand can vary from week applies whatever the state of the market and to week and place to place, and our up-to-date wherever you live: choosing a good agent local knowledge is invaluable for setting prices and marketing your property. improves your odds of a good sale. • We believe that no sale is the same. There’s no one- strategy-fits-all in selling property, and we’ll always Whatever type of property you’re selling, our experienced take the time to listen, learn, discuss and explain team can help you with every aspect of the sale and • We’ll get you started with a free, no-obligation the moving process, from advising you on your pricing appraisal of your property, using our market know- strategy to negotiating the sale to conveyancing. Our how to help you decide if and when to sell. experience and local market know-how will improve your odds of achieving a good price and selling conditions To find out more about how RBS Lindsays can that suit you. help you achieve your home move in 2020, contact our City Centre office to arrange a chat and a free, no-obligation appraisal. Smallburn Steading, Greystone, Carmyllie A unique detached steading conversion with outbuildings located in a peaceful country setting just a few miles from Dundee, Forfar and Arbroath. -
The Place Names of Fife and Kinross
1 n tllif G i* THE PLACE NAMES OF FIFE AND KINROSS THE PLACE NAMES OF FIFE AND KINROSS BY W. J. N. LIDDALL M.A. EDIN., B.A. LOND. , ADVOCATE EDINBURGH WILLIAM GREEN & SONS 1896 TO M. J. G. MACKAY, M.A., LL.D., Advocate, SHERIFF OF FIFE AND KINROSS, AN ACCOMPLISHED WORKER IN THE FIELD OF HISTORICAL RESEARCH. INTRODUCTION The following work has two objects in view. The first is to enable the general reader to acquire a knowledge of the significance of the names of places around him—names he is daily using. A greater interest is popularly taken in this subject than is apt to be supposed, and excellent proof of this is afforded by the existence of the strange corruptions which place names are wont to assume by reason of the effort on the part of people to give some meaning to words otherwise unintelligible to them. The other object of the book is to place the results of the writer's research at the disposal of students of the same subject, or of those sciences, such as history, to which it may be auxiliary. The indisputable conclusion to which an analysis of Fife—and Kinross for this purpose may be considered a Fife— part of place names conducts is, that the nomen- clature of the county may be described as purely of Goidelic origin, that is to say, as belonging to the Irish branch of the Celtic dialects, and as perfectly free from Brythonic admixture. There are a few names of Teutonic origin, but these are, so to speak, accidental to the topography of Fife. -
Scottish Birds
ISSN 0036-9144 SCOTTISH BIRDS THE JOURNAL OF THE SCOTTISH ORNITHOLOGISTS' CLUB Volume 9 No. 4 WINTER 1976 Price 7Sp SCOTTISH BIRD REPORT 1975 1977 SPECIAL INTEREST TOURS by PER'EGRINE HOLIDAYS Director s: Raymond Hodgkins, MA. (Oxon)MTAI. Patricia Hodgkins, MTAI a nd Neville Wykes, (Acct.) All Tours by scheduled Air and Inclusive. Most with guest lecturers and a tour manager. *Provisional SPRING IN VENICE . Mar 19-26 . Art & Leisure £139 SPRING IN ATHENS ... Mar 22-31 . Museums & Leisure £125 SPRING IN ARGOLlS ... Mar 22-31 . Sites & Flowers £152 PELOPONNESE . .. Apr 1-15 ... Birds & Flowers £340 CRETE . Apr 1·15 .. Birds & Flowers £330 MACEDONIA . Apr 28-May 5 . .. Birds with Peter Conder £210 ANDALUSIA .. May 2·14 . Birds & Flowers £220* PELOPONNESE & CRETE ... May 24-Jun 7 . .. Sites & Flowers £345 CRETE (8 days) . , . May 24, 31, June 7 ... Leisure £132 NORTHERN GREECE ... Jun 8·22 ... Mountain Flowers £340 RWANDA & ZAIRE . Jul 15·Aug 3 ... Gorillas with John £898 Gooders. AMAZON & GALAPAGOS . .. Aug 4-24 ... Dr David Bellamy £1064 BIRDS OVER THE BOSPHORUS ... Sep 22-29 ... Eagles with £195 Dr Chris Perrins. KASHMIR & KULU . .. Oct 14-29 ... Birds & Flowers £680* AUTUMN IN ARGOLlS ... Oct 12·21 ... Birds & Sites £153* AUTUMN IN CRETE ... Nov 1-8 ... Birds & Leisure £154* Brochures by return. Registration without obligation. PEREGRINE HOLIDAYS at TOWN AND GOWN TRAVEL, 40/41 SOUTH PARADE, AGENTS SUMMERTOWN, OXFORD, OX2 7JP. Phone Oxford (0865) 511341-2-3 Fully Bonded Atol No. 275B RARE BIRDS IN BRITAIN AND IRELAND by J. T. R. SHARROCKand E. M. SHARROCK This new, much fuller, companion work to Dr Sharrock's Scarce Migrant Birds in Britain and Ireland (£3.80) provides a textual and visual analysis for over 221 species of rare birds seen in these islands. -
Management Statement for Balmerino to Wormit Shore Sssi, Fife
MANAGEMENT STATEMENT FOR BALMERINO TO WORMIT SHORE SSSI, FIFE reviewed 24 April 2008 Contact Address: Scottish Natural Heritage, 46 Crossgate, CUPAR, Fife, KY15 5HS BALMERINO TO WORMIT SHORE SNH Site of Special Scientific Interest 46 Crossgate, Cupar, Fife. KY15 5HS SITE MANAGEMENT STATEMENT Tel: 01334 654038 Fax: 01334 656924 Site Code: 136 e-mail: [email protected] Purpose This is a public statement prepared by SNH for Photo owners and occupiers of the SSSI. It outlines the reasons it is designated as an SSSI and provides guidance on how its special natural features should be conserved or enhanced. This Statement does not affect or form part of the statutory notification and does not remove the need to apply for consent for operations requiring consent. We welcome your views on this statement. Natural features of Condition of feature Balmerino to Wormit Shore SSSI (and date monitored) Old Red Sandstone Igneous Favourable, maintained (October 2006) Description of the site Balmerino to Wormit Shore site of special scientific interest (SSSI) on the southern shore of the Firth of Tay between Balmerino and Wormit, Fife, provides an excellent section through lavas and associated sedimentary rocks which are part of the same group of volcanic rocks that make up the Ochil Hills. These rocks were formed about 410 million years ago, the lavas being erupted from fissures onto low ground or into lakes and rivers. During periods of no volcanic activity sedimentary rocks formed from a mixture of broken lava fragments that were heavily waterworn by the action of rivers that flowed across the surface of the lavas, resulting in pebbles of volcanic origin which were then cemented together into conglomerates by particles of sand deposited in lava cracks. -
Download IF Report Part 1
! Fife Council Review of Onshore Wind Energy in Fife Strategic Cumulative Landscape and Visual Impact Assessment __________________________________________________________________________________________________________________________________________________________________________________________________________ 3.2.2 LandscapeCharacter 9 CONTENTS Page No 3.3 LandscapeDesignations 10 EXECUTIVE SUMMARY 1 3.3.1 NationalLandscapeDesignations 10 1.0 INTRODUCTION 1 3.3.2 LocalLandscapeDesignations 10 1.1 BackgroundtoCumulativeLandscapeIssues 1 3.4 OtherDesignations 11 1.2 ConsultancyAppointments 1 3.4.1 GreenBelts 11 1.3 NationalandLocalPolicy 2 3.4.2 RegionalPark 11 1.4 LandscapeCapacityandCumulativeImpacts 2 3.4.3 HistoricandCulturalDesignations 11 1.5 LimitationsoftheStudy 2 3.4.4 NatureConservationDesignations 12 2.0 METHODOLOGY FOR CUMULATIVE LANDSCAPE AND VISUAL IMPACT AND CAPACITY ASSESSMENT 3 4.0 VISUAL BASELINE 13 2.1 PurposeofMethodology 3 4.1 VisualReceptors 13 2.2 StudyStages 3 4.2 VisibilityAnalysis 13 2.3 ScopeofAssessment 4 4.2.1 Settlements 13 2.3.1 AreaCovered 4 4.2.2 Routes 13 2.3.2 WindEnergyDevelopmentTypes 4 4.2.3 Viewpoints 14 2.4 LandscapeandVisualBaseline 4 4.2.4 AnalysisofVisibility 14 2.5 DeterminingLandscapeSensitivityandCapacity 4 5.0 WIND TURBINES IN THE STUDY AREA 15 2.6 DefiningLandscapeChangeandCumulativeCapacity 5 5.1 TurbineDistribution 15 2.6.1 CumulativeCapacity 5 5.1.1 OperatingandConsentedWindTurbines 15 2.6.2 DeterminingAcceptableLevelsofChange 6 5.1.2 WindfarmApplications