Chapter 2 Ultrashort Carbon Nanotubes
Total Page:16
File Type:pdf, Size:1020Kb
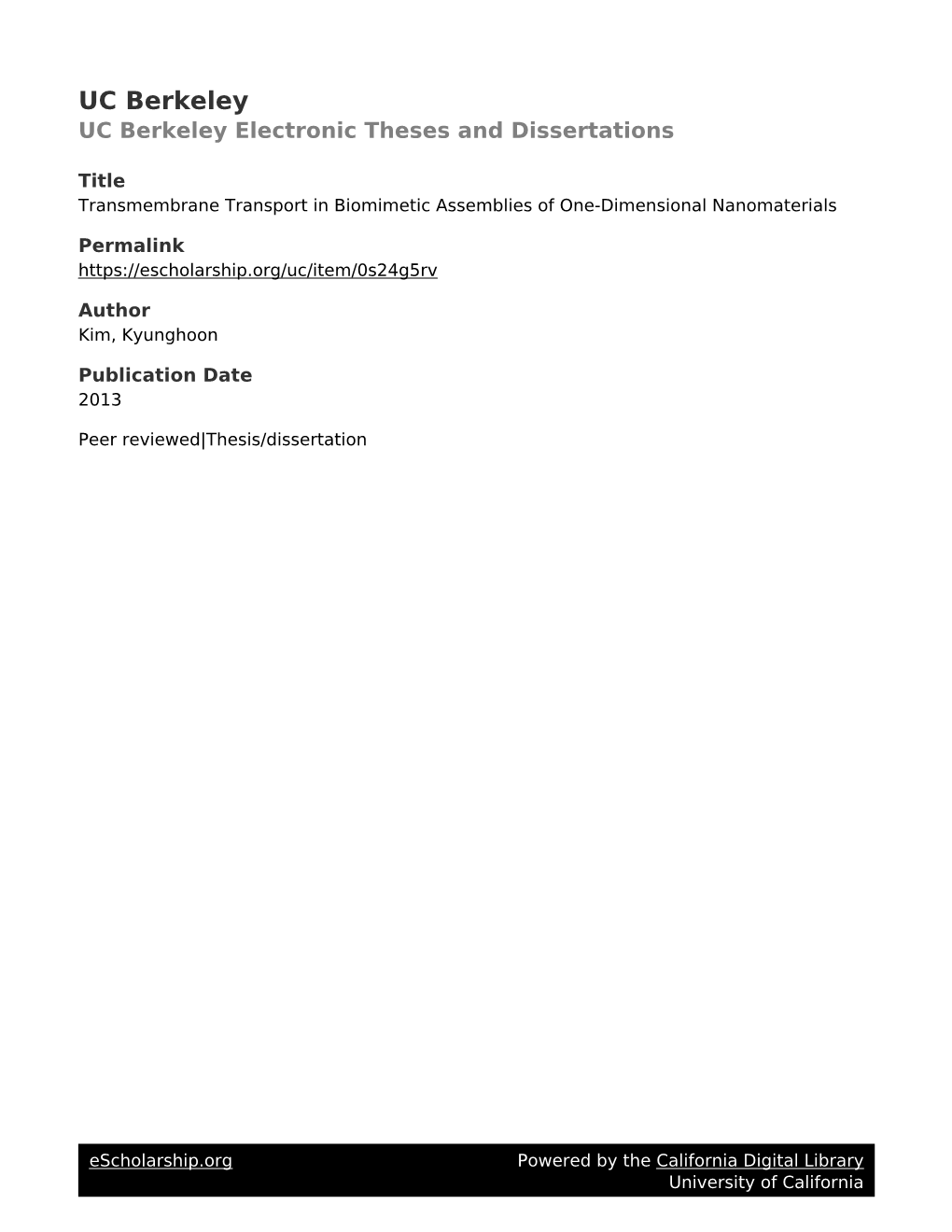
Load more
Recommended publications
-
BBA - Biomembranes 1860 (2018) 566–578
BBA - Biomembranes 1860 (2018) 566–578 Contents lists available at ScienceDirect BBA - Biomembranes journal homepage: www.elsevier.com/locate/bbamem Reconstitution of SNARE proteins into solid-supported lipid bilayer stacks T and X-ray structure analysis Yihui Xua, Jan Kuhlmannb, Martha Brennichc, Karlo Komorowskia, Reinhard Jahnd, Claudia Steinemb, Tim Salditta,* a Institut für Röntgenphysik, Universität Göttingen, Friedrich-Hund-Platz 1, 37077 Göttingen, Germany b Institut für Organische und Biomolekulare Chemie, Universität Göttingen, Tammannstraße 2, Göttingen 37077, Germany c Structural Biology Group, European Synchrotron Radiation Facility, 71 Avenue des Martyrs, CS 90181, Grenoble 38042, France d Department of Neurobiology, Max-Planck Institute for Biophysical Chemistry, Am Faßberg 11, Göttingen 37077, Germany ARTICLE INFO ABSTRACT Keywords: SNAREs are known as an important family of proteins mediating vesicle fusion. For various biophysical studies, SNARE reconstitution they have been reconstituted into supported single bilayers via proteoliposome adsorption and rupture. In this Supported lipid bilayer stack study we extended this method to the reconstitution of SNAREs into supported multilamellar lipid membranes, Micelle and vesicle i.e. oriented multibilayer stacks, as an ideal model system for X-ray structure analysis (X-ray reflectivity and SAXS diffraction). The reconstitution was implemented through a pathway of proteomicelle, proteoliposome and X-ray reflectivity multibilayer. To monitor the structural evolution in each step, we used small-angle X-ray scattering for the GISAXS proteomicelles and proteoliposomes, followed by X-ray reflectivity and grazing-incidence small-angle scattering for the multibilayers. Results show that SNAREs can be successfully reconstituted into supported multibilayers, with high enough orientational alignment for the application of surface sensitive X-ray characterizations. -
Hemifusion and Lateral Lipid Domain Partition in Lipid Membranes of Different Complexity
Dissertation Hemifusion and lateral lipid domain partition in lipid membranes of different complexity zur Erlangung des akademischen Grades doctor rerum naturalium (Dr. rer. nat.) im Fach Biophysik eingereicht an der Mathematisch-Naturwissenschaftlichen Fakultät I der Humboldt-Universität zu Berlin von Diplom Biophysiker Jörg Nikolaus Präsident der Humboldt-Universität zu Berlin Prof. Dr. Jan-Hendrik Olbertz Dekan der Mathematisch-Naturwissenschaftlichen Fakultät I Prof. Dr. Andreas Herrmann Gutachter: 1. Prof. Dr. Andreas Herrmann 2. Prof. Dr. Sandro Keller 3. Prof. Dr. Daniel Huster eingereicht: 16.12.2010 Datum der Promotion: 21.04.2011 „It’s unoptimized - by evolution” Jacob Piehler (* 10.04.1968) Zusammenfassung Die Fusion von Membranen erfordert die Verschmelzung von zwei Phospholipiddoppel- schichten, wobei dies immer über dieselben Zwischenschritte abzulaufen scheint. Eine lokale Störung (‚Stalk’) stellt eine erste Verbindung der äußeren Membranhälften dar, die anschließend lateral expandiert und ein Hemifusionsdiaphragma (HD) bildet. Das Öffnen einer Fusionspore im HD führt zur vollständigen Fusion. Mittels konfokaler Mikroskopie wurde die Fusion von Giant unilamellar vesicles (GUVs) mit negativ geladenen Lipiden und transmembranen (TM) Peptiden in Anwesenheit von zweiwertigen Kationen beobachtet, wobei die Peptide bei der HD Entstehung völlig verdrängt wurden. Eine detaillierte Analyse zeigte, dass es sich bei diesem Mikrometer-großen Bereich um ein HD handelt, dessen Größe von der Lipidzusammensetzung und Peptidkonzentration -
Molecular Mechanism of Fusion Pore Formation Driven by the Neuronal SNARE Complex
Molecular mechanism of fusion pore formation driven by the neuronal SNARE complex Satyan Sharmaa,1 and Manfred Lindaua,b aLaboratory for Nanoscale Cell Biology, Max Planck Institute for Biophysical Chemistry, 37077 Göttingen, Germany and bSchool of Applied and Engineering Physics, Cornell University, Ithaca, NY 14850 Edited by Axel T. Brunger, Stanford University, Stanford, CA, and approved November 1, 2018 (received for review October 2, 2018) Release of neurotransmitters from synaptic vesicles begins with a systems in which various copy numbers of syb2 were incorporated narrow fusion pore, the structure of which remains unresolved. To in an ND while the t-SNAREs were present on a liposome have obtain a structural model of the fusion pore, we performed coarse- been used experimentally to study SNARE-mediated mem- grained molecular dynamics simulations of fusion between a brane fusion (13, 17). The small dimensions of the ND compared nanodisc and a planar bilayer bridged by four partially unzipped with a spherical vesicle makes such systems ideally suited for MD SNARE complexes. The simulations revealed that zipping of SNARE simulations without introducing extreme curvature, which is well complexes pulls the polar C-terminal residues of the synaptobrevin known to strongly influence the propensity of fusion (18–20). 2 and syntaxin 1A transmembrane domains to form a hydrophilic MARTINI-based CGMD simulations have been used in several – core between the two distal leaflets, inducing fusion pore forma- studies of membrane fusion (16, 21 23). To elucidate the fusion tion. The estimated conductances of these fusion pores are in good pore structure and the mechanism of its formation, we performed agreement with experimental values. -
Structural Proteins from Dengue Virus Caracteriz
INSTITUTO DE BIOLOGÍA MOLECULAR Y CELULAR (IBMC) UNIVERSIDAD MIGUEL HERNÁNDEZ DE ELCHE BIOPHYSICAL CHARACTERIZATION OF MEMBRANE- ACTIVE REGIONS OF STRUCTURAL AND NON- STRUCTURAL PROTEINS FROM DENGUE VIRUS CARACTERIZACIÓN BIOFÍSICA DE LAS REGIONES MEMBRANO-ACTIVAS DE PROTEÍNAS ESTRUCTURALES Y NO ESTRUCTURALES DEL VIRUS DEL DENGUE Henrique de Castro Nemésio PhD Thesis / Tesis Doctoral 2014 2 D. Antonio Ferrer Montiel, Catedrático de Bioquímica y Biología Molecular y Celular y Director del Instituto de Biología Molecular y Celular de la Universidad Miguel Hernández de Elche, DA SU CONFORMIDAD a la lectura de tesis doctoral titulada: “Biophysical characterization of membrane-active regions of structural and non- structural proteins from Dengue Virus” / ” Caracterización biofísica de las regiones membrano-activas de proteínas estructurales y no estructurales del virus del Dengue”, para optar al grado de Doctor en Ciencias presentada por D. Henrique de Castro Nemésio. Para que conste y surta los efectos oportunos, firma el presente certificado en Elche, a 25 de Junio de 2014. Fdo. Prof. Antonio Ferrer Montiel 3 4 Don José Villalaín Boullón, Doctor en Ciencias, Investigador del Instituto de Biología Molecular y Celular y Catedrático de Bioquímica y Biología Molecular de la Universidad Miguel Hernández de Elche, CERTIFICA: Que el trabajo de investigación que conduce a la obtención del grado de doctor titulado: “Biophysical characterization of membrane-active regions of structural and non-structural proteins from Dengue Virus” / ” Caracterización biofísica de las regiones membrano-activas de proteínas estructurales y no estructurales del virus del Dengue”, del que es autor D. Henrique de Castro Nemésio, ha sido realizado bajo su dirección en el Instituto de Biología Molecular y Celular de la Universidad Miguel Hernández de Elche. -
Coupling Between Refolding of the Influenza Hemagglutinin and Lipid Rearrangements
1384 Biophysical Journal Volume 75 September 1998 1384–1396 A Mechanism of Protein-Mediated Fusion: Coupling between Refolding of the Influenza Hemagglutinin and Lipid Rearrangements Michael M. Kozlov* and Leonid V. Chernomordik# *Department of Physiology and Pharmacology, Sackler Faculty of Medicine, Tel Aviv University, Ramat Aviv, Tel Aviv 69978, Israel, and #The Laboratory of Cellular and Molecular Biophysics, National Institute of Child Health and Human Development, National Institutes of Health, Bethesda, Maryland 20892 USA ABSTRACT Although membrane fusion mediated by influenza virus hemagglutinin (HA) is the best characterized example of ubiquitous protein-mediated fusion, it is still not known how the low-pH-induced refolding of HA trimers causes fusion. This refolding involves 1) repositioning of the hydrophobic N-terminal sequence of the HA2 subunit of HA (“fusion peptide”), and 2) the recruitment of additional residues to the a-helical coiled coil of a rigid central rod of the trimer. We propose here a mechanism by which these conformational changes can cause local bending of the viral membrane, priming it for fusion. In this model fusion is triggered by incorporation of fusion peptides into viral membrane. Refolding of a central rod exerts forces that pull the fusion peptides, tending to bend the membrane around HA trimer into a saddle-like shape. Elastic energy drives self-assembly of these HA-containing membrane elements in the plane of the membrane into a ring-like cluster. Bulging of the viral membrane within such cluster yields a dimple growing toward the bound target membrane. Bending stresses in the lipidic top of the dimple facilitate membrane fusion. -
Syncytins and Cell Fusion in the Placenta: Structural Insights Into Lipid Membrane Fusion and Placental Development
Syncytins and Cell Fusion in the Placenta: Structural Insights into Lipid Membrane Fusion and Placental Development by Shira Elion-Jourard A thesis submitted in conformity with the requirements for the degree of Master of Science Department of Laboratory Medicine and Pathobiology University of Toronto © Copyright by Shira Elion-Jourard 2018 Syncytins and Cell Fusion in the Placenta: Structural Insights into Lipid Membrane Fusion and Placental Development Shira Elion-Jourard Master of Science Department of Laboratory Medicine and Pathobiology University of Toronto 2018 Abstract Endogenous retroviruses (ERV) are genetic elements found in eukaryotes of retroviral origin. Despite making up a substantial portion of vertebrate genomes, very few ERV genes encode functional proteins. Interestingly, many animal species have evolutionarily converged in the adoption of ERV envelopes for facilitating necessary cell fusions during placental development. These placental ERV envelopes are called syncytins. Structural characterization of syncytins will provide invaluable insight into placental development and evolution as well as contribute to a general understanding of envelope facilitated membrane fusion. Crystallization of and subsequent x-ray crystallographic experiments of human syncytin-2 transmembrane ectodomain in its post-fusion conformation were carried out and the structure was solved at 1.3Å. Comparative analysis between syncytin-2 and other CX6CC type class I viral fusion proteins reveals, despite high structural conservancy, nuanced structural divergences that may reflect functional differences arising by the distinct contexts in which each protein must facilitate membrane fusion. ii Acknowledgments Joining Dr. Jeffrey Lee’s lab almost three years ago, I embarked on a journey in which I explored the world of science and research and learned more about life and myself than I ever could have imagined. -
Direct Observation of Intermediate States in Model Membrane Fusion Andrea Keidel1, Tobias F
www.nature.com/scientificreports OPEN Direct observation of intermediate states in model membrane fusion Andrea Keidel1, Tobias F. Bartsch1,2 & Ernst-Ludwig Florin1 We introduce a novel assay for membrane fusion of solid supported membranes on silica beads and Received: 09 December 2015 on coverslips. Fusion of the lipid bilayers is induced by bringing an optically trapped bead in contact Accepted: 09 March 2016 with the coverslip surface while observing the bead’s thermal motion with microsecond temporal and Published: 31 March 2016 nanometer spatial resolution using a three-dimensional position detector. The probability of fusion is controlled by the membrane tension on the particle. We show that the progression of fusion can be monitored by changes in the three-dimensional position histograms of the bead and in its rate of diffusion. We were able to observe all fusion intermediates including transient fusion, formation of a stalk, hemifusion and the completion of a fusion pore. Fusion intermediates are characterized by axial but not lateral confinement of the motion of the bead and independently by the change of its rate of diffusion due to the additional drag from the stalk-like connection between the two membranes. The detailed information provided by this assay makes it ideally suited for studies of early events in pure lipid bilayer fusion or fusion assisted by fusogenic molecules. Membrane fusion is essential for many life processes. Inside the cell it facilitates exocytosis1,2, protein trafficking3, neurotransmitter release4,5, mitochondrial remodeling6 and resealing of the plasma membrane7. Fusion is a nec- essary step in the life cycle of many viruses8–11. -
Vesicular Stomatitis Virus G Protein Transmembrane Region Is Crucial For
www.nature.com/scientificreports OPEN Vesicular stomatitis virus G protein transmembrane region is crucial for the hemi-fusion to full fusion Received: 9 February 2018 Accepted: 29 June 2018 transition Published: xx xx xxxx Yali Ci1,2, Yang Yang1,2, Caimin Xu1,2 & Lei Shi1,2 Viral fusion proteins are essential for enveloped virus infection. These proteins mediate fusion between the virus envelope and host cellular membrane to release the viral genome into cells. Vesicular stomatitis virus G (VSV G) protein is a typical type III viral fusion protein. To study the mechanism of VSV G protein mediated membrane fusion, we set up a cell-cell fusion system in which cells are marked by diferent fuorescent proteins. Taking advantage of this system, we performed real-time recording and quantitative analysis of the cell fusion mediated by VSV G. We found that the time scale required for VSV G mediated cell-cell fusion was approximately 1–2 minutes. Next, we specifcally examined the function of the transmembrane (TM) region of VSV G protein in membrane fusion by replacing the TM region with those of other fusion proteins. The TM region replacements dramatically impaired VSV G protein function in the cell-cell fusion assay and diminished VSV G mediated lentivirus and recombinant VSV infection efciency. Further experiments implied that the TM region played a role in the transition from hemi-fusion to full fusion. Several residues within the TM region were identifed as important for membrane fusion. Overall, our fndings unraveled the important function of the TM region in VSV G mediated viral fusion. -
Transmembrane Transport in Biomimetic Assemblies of One-Dimensional Nanomaterials
Transmembrane Transport in Biomimetic Assemblies of One-Dimensional Nanomaterials by Kyunghoon Kim A dissertation submitted in partial satisfaction of the requirements for the degree of Doctor of Philosophy in Engineering – Mechanical Engineering in the Graduate Division of the University of California, Berkeley Committee in charge: Professor Costas P. Grigoropoulos, Chair Professor Liwei Lin Professor Seung-Wuk Lee Dr. Aleksandr Noy Fall 2013 Transmembrane Transport in Biomimetic Assemblies of One-Dimensional Nanomaterials Copyright © 2013 By Kyunghoon Kim Abstract Transmembrane Transport in Biomimetic Assemblies of One-Dimensional Nanomaterials by Kyunghoon Kim Doctor of Philosophy in Engineering - Mechanical Engineering University of California, Berkeley Professor Costas P. Grigoropoulos, Chair The creation of biomimetic structures based on one-dimensional nanomaterials and lipid membranes will provide a unique platform for achieving functionalities of biological machines and mimicking nature at the nanoscale. Silicon nanowires (SiNW) and carbon nanotubes (CNT) are of significant interest due to the novel properties not present in bulk materials as well as characteristic dimensions comparable to the size of biological molecules. My thesis describes the creation of fabricated nanomaterials integrated with biomaterials such lipid membranes and their constitutive proteins to create biomimetic assemblies. In the first part of my dissertation, I report transmembrane carbon nanotube pores as a biological ion channel analogs. Biological ion channels in nature transport ions across cellular membranes showing two functions of gating and ion selectivity. CNT pores give structural and functional mimic of an ion channel, in part because smooth, narrow and hydrophobic inner pores of the CNT are remarkably similar to natural biological pores. First, CNTs served as a materials platform that can replicate the features of biological channels. -
PROTEIN-LIPID INTERPLAY in FUSION and FISSION of BIOLOGICAL MEMBRANES Leonid V. Chernomordik1 and Michael M. Kozlov2
Annu. Rev. Biochem. 2003. 72:175–207 doi: 10.1146/annurev.biochem.72.121801.161504 PROTEIN-LIPID INTERPLAY IN FUSION AND * FISSION OF BIOLOGICAL MEMBRANES Leonid V. Chernomordik1 and Michael M. Kozlov2 1Section on Membrane Biology, Laboratory of Cellular and Molecular Biophysics, NICHD, National Institutes of Health, 10 Center Drive, Bethesda, Maryland 20892- 1855; email: [email protected] 2Department of Physiology and Pharmacology, Sackler Faculty of Medicine, Tel Aviv University, Tel Aviv 69978, Israel; email: [email protected] Key Words hemagglutinin, hemifusion, stalk, fusion pore, membrane curvature f Abstract Disparate biological processes involve fusion of two membranes into one and fission of one membrane into two. To formulate the possible job description for the proteins that mediate remodeling of biological membranes, we analyze the energy price of disruption and bending of membrane lipid bilayers at the different stages of bilayer fusion. The phenomenology and the pathways of the well-charac- terized reactions of biological remodeling, such as fusion mediated by influenza hemagglutinin, are compared with those studied for protein-free bilayers. We briefly consider some proteins involved in fusion and fission, and the dependence of remodeling on the lipid composition of the membranes. The specific hypothetical mechanisms by which the proteins can lower the energy price of the bilayer rearrangement are discussed in light of the experimental data and the requirements imposed by the elastic properties of the bilayer. CONTENTS -
Exploring Extracellular Vesicles for Sirna Delivery and Raman-Based Diagnostics
Exploring extracellular vesicles for siRNA delivery and Raman-based diagnostics Stephan Stremersch Pharmacist Master of Science in Drug Development 2016 Thesis submitted to obtain the degree of Doctor in Pharmaceutical Sciences Proefschrift voorgedragen tot het bekomen van de graad van Doctor in de Farmaceutische Wetenschappen Dean Promotor Prof.dr.apr. Jan Van Bocxlaer Prof.dr.apr. Stefaan De Smedt Promotor Promotor Dr.apr. Koen Raemdonck Prof.dr. Kevin Braeckmans Members of the Exam Committee: Prof.dr.apr. Serge Van Calenbergh (chairman) Ghent University Prof.dr.apr. Filip Van Nieuwerburgh (secretary) Ghent University Prof.dr.apr. Olivier De Wever University Hospital Ghent Prof.dr. Raymond Schiffelers University Medical Center Utrecht Prof.dr. Roos Vandenbroucke VIB-Ghent University The author and the promotors give the authorization to consult and to copy parts of this thesis for personal use only. Any other use is limited by the Laws of Copyright, especially the obligation to refer to the source whenever results from this thesis are cited. De auteur en de promotoren geven de toelating dit proefschrift voor consultering beschikbaar te stellen en delen ervan te kopiëren voor persoonlijk gebruik. Elk ander gebruik valt onder de beperkingen van het auteursrecht, in het bijzonder met betrekking tot de verplichting uitdrukkelijk de bron te vermelden bij het aanhalen van resultaten uit dit proefschrift. Ghent, June 24th 2016 The promotors: The author: Prof.dr.apr. Stefaan De Smedt Apr. Stephan Stremersch Dr.apr. Koen Raemdonck Prof.dr. Kevin Braeckmans Table of Contents List of abbreviations 9 Aim and outline of this thesis 13 Chapter 1 Therapeutic and diagnostic applications of extracellular 15 vesicles (EVs) 1. -
Common Principles and Intermediates of Viral Protein-Mediated Fusion: the HIV-1 Paradigm Gregorybmelikyan
Retrovirology BioMed Central Review Open Access Common principles and intermediates of viral protein-mediated fusion: the HIV-1 paradigm GregoryBMelikyan Address: Institute of Human Virology, Department of Microbiology and Immunology, University of Maryland School of Medicine, 725 W. Lombard St, Baltimore, MD 21201, USA Email: Gregory B Melikyan - [email protected] Published: 10 December 2008 Received: 11 November 2008 Accepted: 10 December 2008 Retrovirology 2008, 5:111 doi:10.1186/1742-4690-5-111 This article is available from: http://www.retrovirology.com/content/5/1/111 © 2008 Melikyan; licensee BioMed Central Ltd. This is an Open Access article distributed under the terms of the Creative Commons Attribution License (http://creativecommons.org/licenses/by/2.0), which permits unrestricted use, distribution, and reproduction in any medium, provided the original work is properly cited. Abstract Enveloped viruses encode specialized fusion proteins which promote the merger of viral and cell membranes, permitting the cytosolic release of the viral cores. Understanding the molecular details of this process is essential for antiviral strategies. Recent structural studies revealed a stunning diversity of viral fusion proteins in their native state. In spite of this diversity, the post-fusion structures of these proteins share a common trimeric hairpin motif in which the amino- and carboxy-terminal hydrophobic domains are positioned at the same end of a rod-shaped molecule. The converging hairpin motif, along with biochemical and functional data, implies that disparate viral proteins promote membrane merger via a universal "cast-and-fold" mechanism. According to this model, fusion proteins first anchor themselves to the target membrane through their hydrophobic segments and then fold back, bringing the viral and cellular membranes together and forcing their merger.