Transcriptomic Profiling of Adipose Derived Stem Cells Undergoing
Total Page:16
File Type:pdf, Size:1020Kb
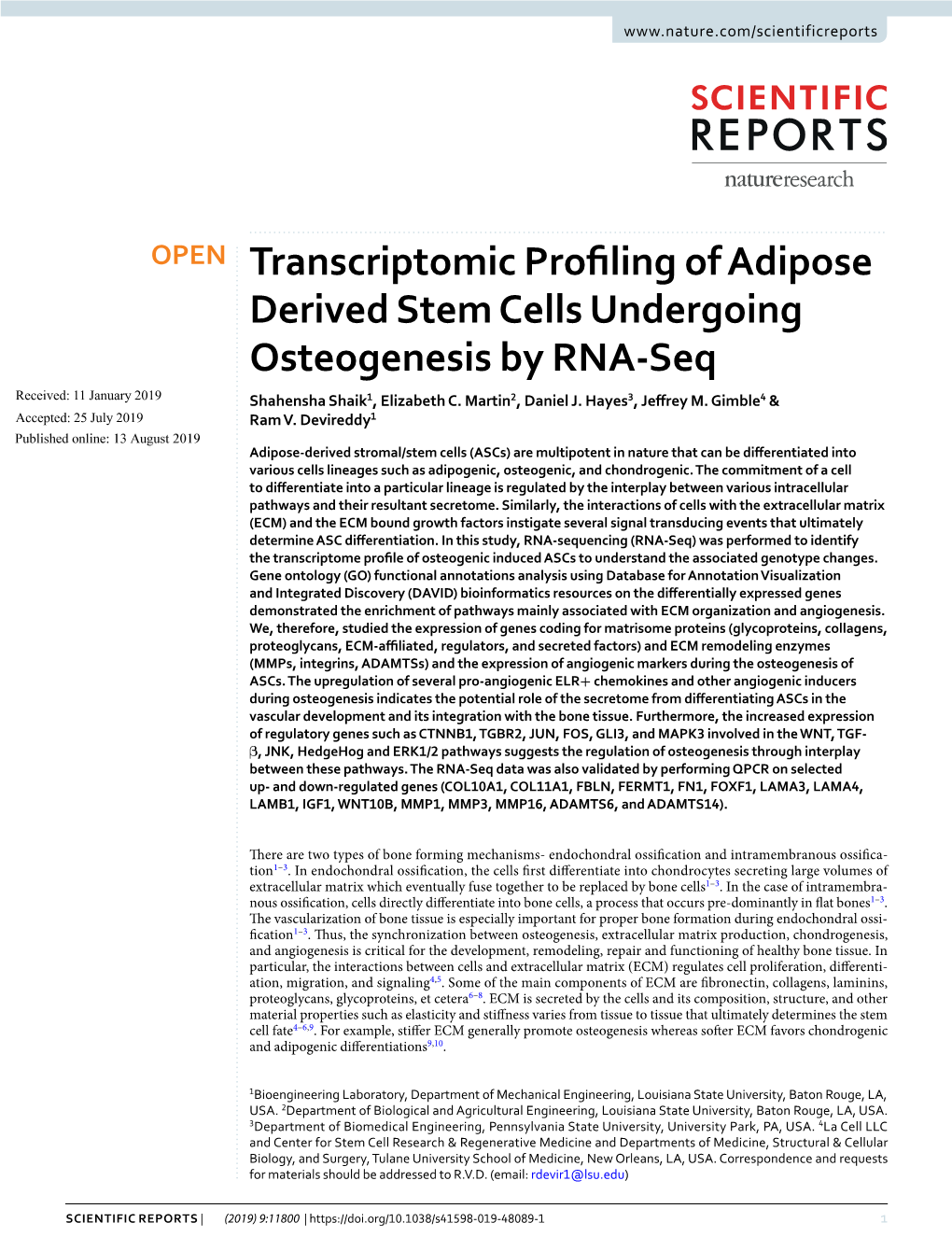
Load more
Recommended publications
-
Supplemental Information to Mammadova-Bach Et Al., “Laminin Α1 Orchestrates VEGFA Functions in the Ecosystem of Colorectal Carcinogenesis”
Supplemental information to Mammadova-Bach et al., “Laminin α1 orchestrates VEGFA functions in the ecosystem of colorectal carcinogenesis” Supplemental material and methods Cloning of the villin-LMα1 vector The plasmid pBS-villin-promoter containing the 3.5 Kb of the murine villin promoter, the first non coding exon, 5.5 kb of the first intron and 15 nucleotides of the second villin exon, was generated by S. Robine (Institut Curie, Paris, France). The EcoRI site in the multi cloning site was destroyed by fill in ligation with T4 polymerase according to the manufacturer`s instructions (New England Biolabs, Ozyme, Saint Quentin en Yvelines, France). Site directed mutagenesis (GeneEditor in vitro Site-Directed Mutagenesis system, Promega, Charbonnières-les-Bains, France) was then used to introduce a BsiWI site before the start codon of the villin coding sequence using the 5’ phosphorylated primer: 5’CCTTCTCCTCTAGGCTCGCGTACGATGACGTCGGACTTGCGG3’. A double strand annealed oligonucleotide, 5’GGCCGGACGCGTGAATTCGTCGACGC3’ and 5’GGCCGCGTCGACGAATTCACGC GTCC3’ containing restriction site for MluI, EcoRI and SalI were inserted in the NotI site (present in the multi cloning site), generating the plasmid pBS-villin-promoter-MES. The SV40 polyA region of the pEGFP plasmid (Clontech, Ozyme, Saint Quentin Yvelines, France) was amplified by PCR using primers 5’GGCGCCTCTAGATCATAATCAGCCATA3’ and 5’GGCGCCCTTAAGATACATTGATGAGTT3’ before subcloning into the pGEMTeasy vector (Promega, Charbonnières-les-Bains, France). After EcoRI digestion, the SV40 polyA fragment was purified with the NucleoSpin Extract II kit (Machery-Nagel, Hoerdt, France) and then subcloned into the EcoRI site of the plasmid pBS-villin-promoter-MES. Site directed mutagenesis was used to introduce a BsiWI site (5’ phosphorylated AGCGCAGGGAGCGGCGGCCGTACGATGCGCGGCAGCGGCACG3’) before the initiation codon and a MluI site (5’ phosphorylated 1 CCCGGGCCTGAGCCCTAAACGCGTGCCAGCCTCTGCCCTTGG3’) after the stop codon in the full length cDNA coding for the mouse LMα1 in the pCIS vector (kindly provided by P. -
University of Groningen Epidermolysis Bullosa Simplex Bolling, Maria
University of Groningen Epidermolysis bullosa simplex Bolling, Maria Caroline IMPORTANT NOTE: You are advised to consult the publisher's version (publisher's PDF) if you wish to cite from it. Please check the document version below. Document Version Publisher's PDF, also known as Version of record Publication date: 2010 Link to publication in University of Groningen/UMCG research database Citation for published version (APA): Bolling, M. C. (2010). Epidermolysis bullosa simplex: new insights in desmosomal cardiocutaneous syndromes. s.n. Copyright Other than for strictly personal use, it is not permitted to download or to forward/distribute the text or part of it without the consent of the author(s) and/or copyright holder(s), unless the work is under an open content license (like Creative Commons). The publication may also be distributed here under the terms of Article 25fa of the Dutch Copyright Act, indicated by the “Taverne” license. More information can be found on the University of Groningen website: https://www.rug.nl/library/open-access/self-archiving-pure/taverne- amendment. Take-down policy If you believe that this document breaches copyright please contact us providing details, and we will remove access to the work immediately and investigate your claim. Downloaded from the University of Groningen/UMCG research database (Pure): http://www.rug.nl/research/portal. For technical reasons the number of authors shown on this cover page is limited to 10 maximum. Download date: 10-10-2021 5 Chromosomal microdeletion explains extracutaneous -
Global Analysis Reveals the Complexity of the Human Glomerular Extracellular Matrix
Global analysis reveals the complexity of the human glomerular extracellular matrix Rachel Lennon,1,2 Adam Byron,1,* Jonathan D. Humphries,1 Michael J. Randles,1,2 Alex Carisey,1 Stephanie Murphy,1,2 David Knight,3 Paul E. Brenchley,2 Roy Zent,4,5 and Martin J. Humphries.1 1Wellcome Trust Centre for Cell-Matrix Research, Faculty of Life Sciences, University of Manchester, Manchester, UK; 2Faculty of Medical and Human Sciences, University of Manchester, Manchester, UK; 3Biological Mass Spectrometry Core Facility, Faculty of Life Sciences, University of Manchester, Manchester, UK; 4Division of Nephrology, Department of Medicine, Vanderbilt University Medical Center, Nashville, TN, USA; and 5Veterans Affairs Hospital, Nashville, TN, USA. *Present address: Edinburgh Cancer Research UK Centre, Institute of Genetics and Molecular Medicine, University of Edinburgh, Edinburgh, UK. Running title: Proteome of the glomerular matrix Word count: Abstract: 208, main text 2765 Corresponding author: Dr Rachel Lennon, Wellcome Trust Centre for Cell-Matrix Research, Michael Smith Building, University of Manchester, Manchester M13 9PT, UK. Phone: 0044 (0) 161 2755498. Fax: 0044 (0) 161 2755082. Email: [email protected] Abstract The glomerulus contains unique cellular and extracellular matrix (ECM) components, which are required for intact barrier function. Studies of the cellular components have helped to build understanding of glomerular disease; however, the full composition and regulation of glomerular ECM remains poorly understood. Here, we employed mass spectrometry–based proteomics of enriched ECM extracts for a global analysis of human glomerular ECM in vivo and identified a tissue-specific proteome of 144 structural and regulatory ECM proteins. This catalogue includes all previously identified glomerular components, plus many new and abundant components. -
Neurogenic Decisions Require a Cell Cycle Independent Function of The
RESEARCH ARTICLE Neurogenic decisions require a cell cycle independent function of the CDC25B phosphatase Fre´ de´ ric Bonnet1†, Angie Molina1†, Me´ lanie Roussat1, Manon Azais2, Sophie Bel-Vialar1, Jacques Gautrais2, Fabienne Pituello1*, Eric Agius1* 1Centre de Biologie du De´veloppement, Centre de Biologie Inte´grative, Universite´ de Toulouse, CNRS, UPS, Toulouse, France; 2Centre de Recherches sur la Cognition Animale, Centre de Biologie Inte´grative., Universite´ de Toulouse, CNRS, UPS, Toulouse, France Abstract A fundamental issue in developmental biology and in organ homeostasis is understanding the molecular mechanisms governing the balance between stem cell maintenance and differentiation into a specific lineage. Accumulating data suggest that cell cycle dynamics play a major role in the regulation of this balance. Here we show that the G2/M cell cycle regulator CDC25B phosphatase is required in mammals to finely tune neuronal production in the neural tube. We show that in chick neural progenitors, CDC25B activity favors fast nuclei departure from the apical surface in early G1, stimulates neurogenic divisions and promotes neuronal differentiation. We design a mathematical model showing that within a limited period of time, cell cycle length modifications cannot account for changes in the ratio of the mode of division. Using a CDC25B point mutation that cannot interact with CDK, we show that part of CDC25B activity is independent *For correspondence: of its action on the cell cycle. [email protected] (FP); [email protected] (EA) †These authors contributed equally to this work Introduction In multicellular organisms, managing the development, homeostasis and regeneration of tissues Competing interests: The requires the tight control of self-renewal and differentiation of stem/progenitor cells. -
Environmental Influences on Endothelial Gene Expression
ENDOTHELIAL CELL GENE EXPRESSION John Matthew Jeff Herbert Supervisors: Prof. Roy Bicknell and Dr. Victoria Heath PhD thesis University of Birmingham August 2012 University of Birmingham Research Archive e-theses repository This unpublished thesis/dissertation is copyright of the author and/or third parties. The intellectual property rights of the author or third parties in respect of this work are as defined by The Copyright Designs and Patents Act 1988 or as modified by any successor legislation. Any use made of information contained in this thesis/dissertation must be in accordance with that legislation and must be properly acknowledged. Further distribution or reproduction in any format is prohibited without the permission of the copyright holder. ABSTRACT Tumour angiogenesis is a vital process in the pathology of tumour development and metastasis. Targeting markers of tumour endothelium provide a means of targeted destruction of a tumours oxygen and nutrient supply via destruction of tumour vasculature, which in turn ultimately leads to beneficial consequences to patients. Although current anti -angiogenic and vascular targeting strategies help patients, more potently in combination with chemo therapy, there is still a need for more tumour endothelial marker discoveries as current treatments have cardiovascular and other side effects. For the first time, the analyses of in-vivo biotinylation of an embryonic system is performed to obtain putative vascular targets. Also for the first time, deep sequencing is applied to freshly isolated tumour and normal endothelial cells from lung, colon and bladder tissues for the identification of pan-vascular-targets. Integration of the proteomic, deep sequencing, public cDNA libraries and microarrays, delivers 5,892 putative vascular targets to the science community. -
2335 Roles of Molecules Involved in Epithelial/Mesenchymal Transition
[Frontiers in Bioscience 13, 2335-2355, January 1, 2008] Roles of molecules involved in epithelial/mesenchymal transition during angiogenesis Giulio Ghersi Dipartimento di Biologia Cellulare e dello Sviluppo, Universita di Palermo, Italy TABLE OF CONTENTS 1. Abstract 2. Introduction 3. Extracellular matrix 3.1. ECM and integrins 3.2. Basal lamina components 4. Cadherins. 4.1. Cadherins in angiogenesis 5. Integrins. 5.1. Integrins in angiogenesis 6. Focal adhesion molecules 7. Proteolytic enzymes 7.1. Proteolytic enzymes inhibitors 7.2. Proteolytic enzymes in angiogenesis 8. Perspective 9. Acknowledgements 10. References 1.ABSTRACT 2. INTRODUCTION Formation of vessels requires “epithelial- Growth of new blood vessels (angiogenesis) mesenchymal” transition of endothelial cells, with several plays a key role in several physiological processes, such modifications at the level of endothelial cell plasma as vascular remodeling during embryogenesis and membranes. These processes are associated with wound healing tissue repair in the adult; as well as redistribution of cell-cell and cell-substrate adhesion pathological processes, including rheumatoid arthritis, molecules, cross talk between external ECM and internal diabetic retinopathy, psoriasis, hemangiomas, and cytoskeleton through focal adhesion molecules and the cancer (1). Vessel formation entails the “epithelial- expression of several proteolytic enzymes, including matrix mesenchymal” transition of endothelial cells (ECs) “in metalloproteases and serine proteases. These enzymes with vivo”; a similar phenotypic exchange can be induced “in their degradative action on ECM components, generate vitro” by growing ECs to low cell density, or in “wound molecules acting as activators and/or inhibitors of healing” experiments or perturbing cell adhesion and angiogenesis. The purpose of this review is to provide an associated molecule functions. -
Comparative Transcriptome Analysis of Embryo Invasion in the Mink Uterus
Placenta 75 (2019) 16–22 Contents lists available at ScienceDirect Placenta journal homepage: www.elsevier.com/locate/placenta Comparative transcriptome analysis of embryo invasion in the mink uterus T ∗ Xinyan Caoa,b, , Chao Xua,b, Yufei Zhanga,b, Haijun Weia,b, Yong Liuc, Junguo Caoa,b, Weigang Zhaoa,b, Kun Baoa,b, Qiong Wua,b a Institute of Special Animal and Plant Sciences, Chinese Academy of Agricultural Sciences, Changchun, China b State Key Laboratory for Molecular Biology of Special Economic Animal and Plant Science, Chinese Academy of Agricultural Sciences, Changchun, China c Key Laboratory of Embryo Development and Reproductive Regulation of Anhui Province, College of Biological and Food Engineering, Fuyang Teachers College, Fuyang, China ABSTRACT Introduction: In mink, as many as 65% of embryos die during gestation. The causes and the mechanisms of embryonic mortality remain unclear. The purpose of our study was to examine global gene expression changes during embryo invasion in mink, and thereby to identify potential signaling pathways involved in implantation failure and early pregnancy loss. Methods: Illumina's next-generation sequencing technology (RNA-Seq) was used to analyze the differentially expressed genes (DEGs) in implantation (IMs) and inter- implantation sites (inter-IMs) of uterine tissue. Results: We identified a total of 606 DEGs, including 420 up- and 186 down-regulated genes in IMs compared to inter-IMs. Gene annotation analysis indicated multiple biological pathways to be significantly enriched for DEGs, including immune response, ECM complex, cytokine activity, chemokine activity andprotein binding. The KEGG pathway including cytokine-cytokine receptor interaction, Jak-STAT, TNF and the chemokine signaling pathway were the most enriched. -
Three Dact Gene Family Members Are Expressed During Embryonic Development
Three Dact Gene Family Members are Expressed During Embryonic Development and in the Adult Brains of Mice Daniel A Fisher, Saul Kivimäe, Jun Hoshino, Rowena Suriben, Pierre -Marie Martin, Nichol Baxter, Benjamin NR Cheyette Department of Psychiatry & Gra duate Programs in Developmental Biology and Neuroscience, University of California, San Francisco, 94143-2611 Correspondence: Benjamin NR Cheyette [email protected] 415.476.7826 Running Title: Mouse Dact Gene Family Expression Key Words: mouse, Dpr, Frodo, Thyex, Dact, Wnt, Dvl, expression, embryo, brain Supported by: NIH: MH01750 K08; NARSAD Young Investigator Award; NAAR award #551. Abstract Members of the Dact protein family were initially identified through binding to Dishevelled (Dvl), a cytoplasmic protein central to Wnt signaling. During mouse development, Dact1 is detected in the presomitic mesoderm and somites during segmentation, in the limb bud mesenchyme and other mesoderm-derived tissues, and in the central nervous system (CNS). Dact2 expression is most prominent during organogenesis of the thymus, kidneys, and salivary glands, with much lower levels in the somites and in the developing CNS. Dact3, not previously described in any organism, is expressed in the ventral region of maturing somites, limb bud and branchial arch mesenchyme, and in the embryonic CNS; of the three paralogs it is the most highly expressed in the adult cerebral cortex. These data are consistent with studies in other vertebrates showing that Dact paralogs have distinct signaling and developmental roles, and suggest they may differentially contribute to postnatal brain physiology. Introduction Signaling downstream of secreted Wnt ligands is a conserved process in multicellular animals that plays important roles during development and, when misregulated, contributes to cancer and other diseases (Polakis, 2000; Moon et al., 2002). -
ADAMTS13 and 15 Are Not Regulated by the Full Length and N‑Terminal Domain Forms of TIMP‑1, ‑2, ‑3 and ‑4
BIOMEDICAL REPORTS 4: 73-78, 2016 ADAMTS13 and 15 are not regulated by the full length and N‑terminal domain forms of TIMP‑1, ‑2, ‑3 and ‑4 CENQI GUO, ANASTASIA TSIGKOU and MENG HUEE LEE Department of Biological Sciences, Xian Jiaotong-Liverpool University, Suzhou, Jiangsu 215123, P.R. China Received June 29, 2015; Accepted July 15, 2015 DOI: 10.3892/br.2015.535 Abstract. A disintegrin and metalloproteinase with thom- proteolysis activities associated with arthritis, morphogenesis, bospondin motifs (ADAMTS) 13 and 15 are secreted zinc angiogenesis and even ovulation [as reviewed previously (1,2)]. proteinases involved in the turnover of von Willebrand factor Also known as the VWF-cleaving protease, ADAMTS13 and cancer suppression. In the present study, ADAMTS13 is noted for its ability in cleaving and reducing the size of the and 15 were subjected to inhibition studies with the full-length ultra-large (UL) form of the VWF. Reduction in ADAMTS13 and N-terminal domain forms of tissue inhibitor of metallo- activity from either hereditary or acquired deficiency causes proteinases (TIMPs)-1 to -4. TIMPs have no ability to inhibit accumulation of UL-VWF multimers, platelet aggregation and the ADAMTS proteinases in the full-length or N-terminal arterial thrombosis that leads to fatal thrombotic thrombocy- domain form. While ADAMTS13 is also not sensitive to the topenic purpura [as reviewed previously (1,3)]. By contrast, hydroxamate inhibitors, batimastat and ilomastat, ADAMTS15 ADAMTS15 is a potential tumor suppressor. Only a limited app can be effectively inhibited by batimastat (Ki 299 nM). In number of in-depth investigations have been carried out on the conclusion, the present results indicate that TIMPs are not the enzyme; however, expression and profiling studies have shown regulators of these two ADAMTS proteinases. -
Systematic Cpg Islands Methylation Profiling of Genes in the Wnt Pathway in Epithelial Ovarian Cancer Identifies Biomarkers of Progression-Free Survival
Published OnlineFirst April 1, 2011; DOI: 10.1158/1078-0432.CCR-10-3021 Clinical Cancer Imaging, Diagnosis, Prognosis Research Systematic CpG Islands Methylation Profiling of Genes in the Wnt Pathway in Epithelial Ovarian Cancer Identifies Biomarkers of Progression-Free Survival Wei Dai1, Jens M. Teodoridis1, Constanze Zeller1, Janet Graham1, Jenny Hersey3, James M. Flanagan1, Euan Stronach2, David W. Millan4, Nadeem Siddiqui5, Jim Paul6, and Robert Brown1,3 Abstract Purpose: Wnt pathways control key biological processes that potentially impact on tumor progression and patient survival. We aimed to evaluate DNA methylation at promoter CpG islands (CGI) of Wnt pathway genes in ovarian tumors at presentation and identify biomarkers of patient progression-free survival (PFS). Experimental Design: Epithelial ovarian tumors (screening study n ¼ 120, validation study n ¼ 61), prospectively collected through a cohort study, were analyzed by differential methylation hybridization at 302 loci spanning 189 promoter CGIs at 137 genes in Wnt pathways. The association of methylation and PFS was examined by Cox proportional hazards model. Results: DNA methylation is associated with PFS at 20 of 302 loci (P < 0.05, n ¼ 111), with 5 loci significant at false discovery rate (FDR) less than 10%. A total of 11 of 20 loci retain significance in an independent validation cohort (n ¼ 48, P 0.05, FDR 10%), and 7 of these loci, at FZD4, DVL1, NFATC3, ROCK1, LRP5, AXIN1, and NKD1 genes, are independent from clinical parameters (adjusted P < 0.05). Increased methylation at these loci associates with increased hazard of disease progression. A multivariate Cox model incorporates only NKD1 and DVL1, identifying two groups differing in PFS [HR ¼ 2.09; 95% CI (1.39–3.15); permutation test P < 0.005]. -
Supplementary Table 1: Adhesion Genes Data Set
Supplementary Table 1: Adhesion genes data set PROBE Entrez Gene ID Celera Gene ID Gene_Symbol Gene_Name 160832 1 hCG201364.3 A1BG alpha-1-B glycoprotein 223658 1 hCG201364.3 A1BG alpha-1-B glycoprotein 212988 102 hCG40040.3 ADAM10 ADAM metallopeptidase domain 10 133411 4185 hCG28232.2 ADAM11 ADAM metallopeptidase domain 11 110695 8038 hCG40937.4 ADAM12 ADAM metallopeptidase domain 12 (meltrin alpha) 195222 8038 hCG40937.4 ADAM12 ADAM metallopeptidase domain 12 (meltrin alpha) 165344 8751 hCG20021.3 ADAM15 ADAM metallopeptidase domain 15 (metargidin) 189065 6868 null ADAM17 ADAM metallopeptidase domain 17 (tumor necrosis factor, alpha, converting enzyme) 108119 8728 hCG15398.4 ADAM19 ADAM metallopeptidase domain 19 (meltrin beta) 117763 8748 hCG20675.3 ADAM20 ADAM metallopeptidase domain 20 126448 8747 hCG1785634.2 ADAM21 ADAM metallopeptidase domain 21 208981 8747 hCG1785634.2|hCG2042897 ADAM21 ADAM metallopeptidase domain 21 180903 53616 hCG17212.4 ADAM22 ADAM metallopeptidase domain 22 177272 8745 hCG1811623.1 ADAM23 ADAM metallopeptidase domain 23 102384 10863 hCG1818505.1 ADAM28 ADAM metallopeptidase domain 28 119968 11086 hCG1786734.2 ADAM29 ADAM metallopeptidase domain 29 205542 11085 hCG1997196.1 ADAM30 ADAM metallopeptidase domain 30 148417 80332 hCG39255.4 ADAM33 ADAM metallopeptidase domain 33 140492 8756 hCG1789002.2 ADAM7 ADAM metallopeptidase domain 7 122603 101 hCG1816947.1 ADAM8 ADAM metallopeptidase domain 8 183965 8754 hCG1996391 ADAM9 ADAM metallopeptidase domain 9 (meltrin gamma) 129974 27299 hCG15447.3 ADAMDEC1 ADAM-like, -
Novel Pathogenic Mutations of FERMT1 in Two Chinese Kindler Syndrome Families
Novel Pathogenic Mutations of FERMT1 in two Chinese Kindler Syndrome Families Min Li First Aliated Hospital of Soochow University Weisheng Li First Aliated Hospital of Soochow University Dan Zhu First Aliated Hospital of Soochow University Likui Lu First Aliated Hospital of Soochow University Jingliu Liu First Aliated Hospital of Soochow University Yajun Shi First Aliated Hospital of Soochow University Zhimiao Lin Peking University First Hospital Miao Sun ( [email protected] ) First Aliated Hospital of Soochow University https://orcid.org/0000-0002-6414-368X Research Keywords: Kindler syndrome, FERMT1, kindlin-1, nonsense mutation, frame-shift mutation Posted Date: March 31st, 2021 DOI: https://doi.org/10.21203/rs.3.rs-354474/v1 License: This work is licensed under a Creative Commons Attribution 4.0 International License. Read Full License Page 1/13 Abstract Background: Kindler syndrome (KNDLRS) is a very rare autosomal recessive disorder characterized by bullous poikiloderma with photosensitivity. Loss-of-function mutations in FERMT1, which located on chromosome 20p12.3, were responsible for KNDLRS. Numerous mutations in FERMT1 have been reported to be associated with KNDLRS. Results: The present study reported two Chinese KNDLRS families, and affected individuals from both families presented with poikiloderma, palmoplantar hyperkeratosis, and diffuse cigarette paper like atrophy on hands. Skin biopsy of the proband from family 2 showed atrophy of epidermis, hyperkeratosis, dilated blood vessels in upper dermis, and microbubbles at the dermis and epidermis junction. Medical Whole Exome Sequencing V4 combined with Sanger sequencing revealed mutations in FERMT1 with affected individuals. Compound heterozygous nonsense mutations (c.193C>T, c.277C>T) were found with family 1, and a homozygous frameshift mutation (c.220delC) was observed in family 2.