Sex Determination Using RNA-Sequencing Analyses in Early Prenatal Pig Development
Total Page:16
File Type:pdf, Size:1020Kb
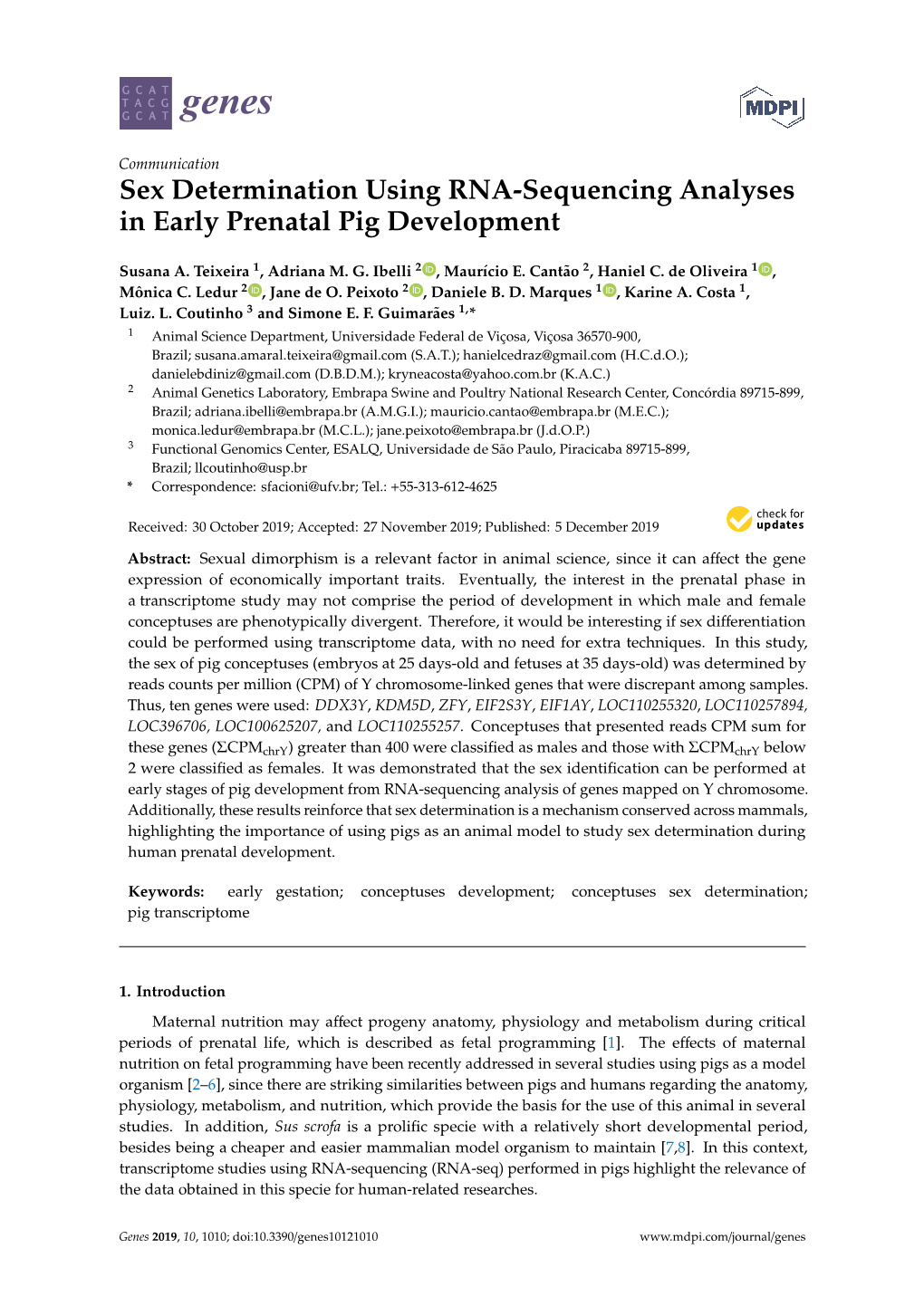
Load more
Recommended publications
-
Network Medicine Approach for Analysis of Alzheimer's Disease Gene Expression Data
International Journal of Molecular Sciences Article Network Medicine Approach for Analysis of Alzheimer’s Disease Gene Expression Data David Cohen y, Alexander Pilozzi y and Xudong Huang * Neurochemistry Laboratory, Department of Psychiatry, Massachusetts General Hospital and Harvard Medical School, Charlestown, MA 02129, USA; [email protected] (D.C.); [email protected] (A.P.) * Correspondence: [email protected]; Tel./Fax: +1-617-724-9778 These authors contributed equally to this work. y Received: 15 November 2019; Accepted: 30 December 2019; Published: 3 January 2020 Abstract: Alzheimer’s disease (AD) is the most widespread diagnosed cause of dementia in the elderly. It is a progressive neurodegenerative disease that causes memory loss as well as other detrimental symptoms that are ultimately fatal. Due to the urgent nature of this disease, and the current lack of success in treatment and prevention, it is vital that different methods and approaches are applied to its study in order to better understand its underlying mechanisms. To this end, we have conducted network-based gene co-expression analysis on data from the Alzheimer’s Disease Neuroimaging Initiative (ADNI) database. By processing and filtering gene expression data taken from the blood samples of subjects with varying disease states and constructing networks based on that data to evaluate gene relationships, we have been able to learn about gene expression correlated with the disease, and we have identified several areas of potential research interest. Keywords: Alzheimer’s disease; network medicine; gene expression; neurodegeneration; neuroinflammation 1. Introduction Alzheimer’s disease (AD) is the most widespread diagnosed cause of dementia in the elderly [1]. -
The Role of the X Chromosome in Embryonic and Postnatal Growth
The role of the X chromosome in embryonic and postnatal growth Daniel Mark Snell A dissertation submitted in partial fulfillment of the requirements for the degree of Doctor of Philosophy of University College London. Francis Crick Institute/Medical Research Council National Institute for Medical Research University College London January 28, 2018 2 I, Daniel Mark Snell, confirm that the work presented in this thesis is my own. Where information has been derived from other sources, I confirm that this has been indicated in the work. Abstract Women born with only a single X chromosome (XO) have Turner syndrome (TS); and they are invariably of short stature. XO female mice are also small: during embryogenesis, female mice with a paternally-inherited X chromosome (XPO) are smaller than XX littermates; whereas during early postnatal life, both XPO and XMO (maternal) mice are smaller than their XX siblings. Here I look to further understand the genetic bases of these phenotypes, and potentially inform areas of future investigation into TS. Mouse pre-implantation embryos preferentially silence the XP via the non-coding RNA Xist.XPO embryos are smaller than XX littermates at embryonic day (E) 10.5, whereas XMO embryos are not. Two possible hypotheses explain this obser- vation. Inappropriate expression of Xist in XPO embryos may cause transcriptional silencing of the single X chromosome and result in embryos nullizygous for X gene products. Alternatively, there could be imprinted genes on the X chromosome that impact on growth and manifest in growth retarded XPO embryos. In contrast, dur- ing the first three weeks of postnatal development, both XPO and XMO mice show a growth deficit when compared with XX littermates. -
Zfyl Encodes a Nuclear Sequence-Specific DNA Binding
View metadata, citation and similar papers at core.ac.uk brought to you byCORE provided by Elsevier - Publisher Connector FEBS 15213 FEBS Letters 360 (1995) 315-319 Zfyl encodes a nuclear sequence-specific DNA binding protein Pamela Taylor-Harris, Sally Swift, Alan Ashworth* CRC Centre of Cell and Molecular Biology, Chester Beatty Laboratories, The Institute of Cancer Research, 237 Fulham Road, London, SW3 6JB, UK Received 19 January 1995; revised version received 3 February 1995 sively to RNA and have functions in RNA storage or processes Abstract Zfyl is a mouse Y chromosomal gene encoding a zinc such as splicing. finger protein which is thought to have some function during In order to analyse the properties of the ZFY family of zinc spermatogenesis. Here we show that, when introduced into tissue finger proteins, we have raised specific antibodies to murine culture cells, Zfyl is targeted to the nucleus. Two independent signals are present within the protein for nuclear localization. Zfyl. These have been used to demonstrate that Zfyl protein This nuclear Zfyl protein is able to bind strongly to DNA-ceHu- localizes to the nucleus of transfected cells and to identify spe- lose and, using site-selection assays, we have identified specific cific DNA binding sites for Zfyl, suggesting a role for this Zfyl DNA binding sites. Taken together these results suggest protein as a transcription factor regulating gene expression that Zfyl is a nuclear-located sequence-specific DNA binding during spermatogenesis. protein which functions during spermatogenesis. Key words: Spermatogenesis; Zinc finger protein; 2. Materials and methods Y chromosome; Nucleus; DNA binding; Transcription factor 2.1. -
Downloaded from Interpro Database (IPR013087)
bioRxiv preprint doi: https://doi.org/10.1101/637298; this version posted May 15, 2019. The copyright holder for this preprint (which was not certified by peer review) is the author/funder, who has granted bioRxiv a license to display the preprint in perpetuity. It is made available under aCC-BY-NC 4.0 International license. Why Do Long Zinc Finger Proteins have Short Motifs? A case study of ZFY and CTCF reveals non-independent recognition of tandem zinc finger proteins. Zheng Zuo1*, Timothy Billings6, Michael Walker6, Petko Petkov6, Polly Fordyce1, 2, 3, 4, Gary D. Stormo5* 1. Department of Genetics, Stanford University, CA, USA 2. Chan Zuckerberg Biohub, San Francisco, CA, USA 3. Department of Bioengineering, Stanford University, CA, USA 4. Stanford CheM-H Institute, Stanford University, CA, USA 5. Department of Genetics, Washington University in St. Louis, MO, USA 6. The Jackson Laboratory, ME, USA Correspondence: [email protected] Summary The human genome has more than 800 C2H2 Zinc Finger-containing genes, and many of them are composed of long tandem arrays of zinc fingers. Current Zinc Finger Protein (ZFP) motif prediction models assume longer finger arrays correspond to longer DNA-binding motifs and higher specificity. However, recent experimental efforts to identify ZFP binding sites in vivo contradict this assumption with many having short reported motifs. Using Zinc Finger Y (ZFY), which has 13 ZFs, we quantitatively characterize its DNA binding specificity with several complementary methods, including Affinity-seq, HT-SELEX, Spec-seq and fluorescence anisotropy. Besides the previously identified core motif GGCCT recognized by fingers 12-13, we find a novel secondary irregular motif recognized by accessory fingers. -
A Genetic Method for Sex Identification of Raccoons (Procyon Lotor) with Using the ZFX and ZFY Genes
NOTE Wildlife Science A Genetic Method for Sex Identification of Raccoons (Procyon lotor) with Using the ZFX and ZFY Genes Minami W. OKUYAMA1), Michito SHIMOZURU1) and Toshio TSUBOTA1)* 1)Laboratory of Wildlife Biology and Medicine, Graduate School of Veterinary Medicine, Hokkaido University, Kita18, Nichi9, Kita-ku, Sapporo, Hokkaido 060–0818, Japan (Received 19 November 2013/Accepted 2 January 2014/Published online in J-STAGE 23 January 2014) ABSTRACT. A genetic method for sex determination in raccoons was developed based on nucleotide differences of the zinc finger protein genes ZFX and ZFY. Four novel internal primers specific for ZFX or ZFY were designed. PCR amplification using two primer sets followed by agarose gel electrophoresis enabled sex determination. 141-bp and 447-bp bands were in both sex, and 346-bp band was specific only in male with primer set I. 345-bp and 447-bp bands were in both sex, and 141-bp band was specific only in male with primer set II, which could distinguish raccoon’s electrophoresis pattern from three native carnivores in Hokkaido. This method will be useful for conservation genetics studies or biological analyses of raccoons. KEY WORDS: PCR, raccoons, sex identification, ZFX and ZFY genes. doi: 10.1292/jvms.13-0577; J. Vet. Med. Sci. 76(5): 773–775, 2014 Sex is one of the most important pieces of information between ZFX and ZFY in raccoons and to establish a new about an animal, as it is related to physiology, behavior and genetic method for sex determination of raccoons. reproduction. Thus, developing methods for sex identifica- Hair or whisker samples were collected from the carcasses tion are essential in many fields of study, including zoology of feral raccoons that were euthanized for eradication control and ecology. -
Normal Structure and Expression of Zfy Genes in XY Female Mice Mutant in Tdy
Development 109, 647-653 (1990) 647 Printed in Great Britain ©The Company of Biologists Limited 1990 Normal structure and expression of Zfy genes in XY female mice mutant in Tdy JOHN GUBBAY1, PETER KOOPMAN1, JEROME COLLIGNON1, PAUL BURGOYNE2 and ROBIN LOVELL-BADGE1* [ Laboratory of Eukaryotic Molecular Genetics, National Institute for Medical Research, The Ridgeway, Mill Hill, London NW71AA, UK 2MRC Mammalian Development Unit, Wolfson House, 4 Stephenson Way, London NWI 2HE, UK •To whom correspondence should be addressed Summary Zfy-1 and Zfy-2 are candidate genes for Tdy, the testis- show a normal structure and expression pattern in mice determining gene in mice. We have analysed these genes with a Y chromosome known to carry a mutation in Tdy in a line of XY female mice that have been shown to be and that mutant embryos develop into females despite mutated in Tdy. We have used Southern blot analysis to Zfy-1 expression, strongly supports other recent evi- show that the Zfy genes have not undergone any major dence that Zfy genes are not directly involved in primary structural alterations, and have also demonstrated that testis determination. both genes are transcribed normally from the mutant Y chromosome (¥) in both adult XY¥ testis and X¥ Key words: sex determination, Tdy, Zfy, zinc finger genes, female embryonic gonads. The fact that these genes polymerase chain reaction, gene expression. Introduction conservation and cell autonomous action of the testis- determining gene (Burgoyne et al. 1988). Sex determination in mammals is dependent upon the However, theories of the mode of action of ZFY in action of a Y-linked testis-determining gene termed testis determination have to take into account the TDF in humans and Tdy in mice (Goodfellow and presence of a highly homologous gene (ZFX) present Darling, 1988; McLaren, 1988). -
Prenatal Sex Differences in the Human Brain
Molecular Psychiatry (2009) 14, 988–991 & 2009 Nature Publishing Group All rights reserved 1359-4184/09 $32.00 www.nature.com/mp LETTERS TO THE EDITOR Prenatal sex differences in the human brain Molecular Psychiatry (2009) 14, 988–989. doi:10.1038/ development. These genes are not only expressed in mp.2009.79 the brain before birth but some of them are also known to have sex differences in adult brain,1,4 whereas others are expressed during infancy, but The presence of genetic sex differences in the adult reduced later on during their lifetime.5 human brain is now recognized.1 We hypothesized Intriguingly, SRY, a well-known determinant of that the basis of this sex bias is already established in testicle development during midgestation,6 showed the brain before birth. Here, we show that several no evidence of expression in any of the brain regions genes encoded in the Y-chromosome are expressed in analyzed (Figure 1b, and Supplementary Figure 1), many regions of the male prenatal brain, likely having suggesting that the main somatic sex determinants functional consequences for sex bias during human may be different for the brain and gonads during brain development. human gestation. The marked sex differences in age at onset, In humans, all 11 genes described here are encoded prevalence and symptoms for numerous neuropsy- in the male-specific region of the Y-chromosome,7 chiatric disorders2 indicate the importance to study with RPS4Y1 and ZFY located in the p-arm very close the emergence of a sex bias during human brain to SRY and most of the remaining genes located in the development. -
Quantitative Analysis of Y-Chromosome Gene Expression Across 36 Human Tissues 6 7 8 9 Alexander K
Downloaded from genome.cshlp.org on September 26, 2021 - Published by Cold Spring Harbor Laboratory Press 1 2 3 4 5 Quantitative analysis of Y-Chromosome gene expression across 36 human tissues 6 7 8 9 Alexander K. Godfrey1,2, Sahin Naqvi1,2, Lukáš Chmátal1, Joel M. Chick3, 10 Richard N. Mitchell4, Steven P. Gygi3, Helen Skaletsky1,5, David C. Page1,2,5* 11 12 13 1 Whitehead Institute, Cambridge, MA, USA 14 2 Department of Biology, Massachusetts Institute of Technology, Cambridge, MA, USA 15 3 Department of Cell Biology, Harvard Medical School, Boston, MA, USA 16 4 Department of Pathology, Brigham and Women’s Hospital, Harvard Medical School, Boston, MA, USA 17 5 Howard Hughes Medical Institute, Whitehead Institute, Cambridge, MA, USA 18 19 20 21 *corresponding author: 22 Email: [email protected] 23 24 25 Running title: 26 Human Y-Chromosome gene expression in 36 tissues 27 28 29 Keywords: 30 Y Chromosome, sex chromosomes, sex differences, EIF1AY, EIF1AX 31 Downloaded from genome.cshlp.org on September 26, 2021 - Published by Cold Spring Harbor Laboratory Press 32 ABSTRACT 33 Little is known about how human Y-Chromosome gene expression directly contributes to 34 differences between XX (female) and XY (male) individuals in non-reproductive tissues. Here, 35 we analyzed quantitative profiles of Y-Chromosome gene expression across 36 human tissues 36 from hundreds of individuals. Although it is often said that Y-Chromosome genes are lowly 37 expressed outside the testis, we report many instances of elevated Y-Chromosome gene 38 expression in a non-reproductive tissue. -
Genetics of Azoospermia
International Journal of Molecular Sciences Review Genetics of Azoospermia Francesca Cioppi , Viktoria Rosta and Csilla Krausz * Department of Biochemical, Experimental and Clinical Sciences “Mario Serio”, University of Florence, 50139 Florence, Italy; francesca.cioppi@unifi.it (F.C.); viktoria.rosta@unifi.it (V.R.) * Correspondence: csilla.krausz@unifi.it Abstract: Azoospermia affects 1% of men, and it can be due to: (i) hypothalamic-pituitary dysfunction, (ii) primary quantitative spermatogenic disturbances, (iii) urogenital duct obstruction. Known genetic factors contribute to all these categories, and genetic testing is part of the routine diagnostic workup of azoospermic men. The diagnostic yield of genetic tests in azoospermia is different in the different etiological categories, with the highest in Congenital Bilateral Absence of Vas Deferens (90%) and the lowest in Non-Obstructive Azoospermia (NOA) due to primary testicular failure (~30%). Whole- Exome Sequencing allowed the discovery of an increasing number of monogenic defects of NOA with a current list of 38 candidate genes. These genes are of potential clinical relevance for future gene panel-based screening. We classified these genes according to the associated-testicular histology underlying the NOA phenotype. The validation and the discovery of novel NOA genes will radically improve patient management. Interestingly, approximately 37% of candidate genes are shared in human male and female gonadal failure, implying that genetic counselling should be extended also to female family members of NOA patients. Keywords: azoospermia; infertility; genetics; exome; NGS; NOA; Klinefelter syndrome; Y chromosome microdeletions; CBAVD; congenital hypogonadotropic hypogonadism Citation: Cioppi, F.; Rosta, V.; Krausz, C. Genetics of Azoospermia. 1. Introduction Int. J. Mol. Sci. -
Distinct Prophase Arrest Mechanisms in Human Male Meiosis
bioRxiv preprint doi: https://doi.org/10.1101/195321; this version posted September 28, 2017. The copyright holder for this preprint (which was not certified by peer review) is the author/funder. All rights reserved. No reuse allowed without permission. Distinct prophase arrest mechanisms in human male meiosis Sabrina Z. Jan1, Aldo Jongejan2, Cindy M. Korver1, Saskia K.M. van Daalen1, Ans M.M. van Pelt1, Sjoerd Repping1 and Geert Hamer1,* 1 Center for Reproductive Medicine, Amsterdam Research Institute Reproduction and Development, Academic Medical Center, University of Amsterdam, Amsterdam, The Netherlands 2 Bioinformatics Laboratory, Department of Clinical Epidemiology, Biostatistics and Bioinformatics, Academic Medical Center Amsterdam, The Netherlands * Correspondence: [email protected] 1 bioRxiv preprint doi: https://doi.org/10.1101/195321; this version posted September 28, 2017. The copyright holder for this preprint (which was not certified by peer review) is the author/funder. All rights reserved. No reuse allowed without permission. To prevent chromosomal aberrations to be transmitted to the offspring, strict meiotic checkpoints are in place to remove aberrant spermatocytes. However, in about 1% of all males these checkpoints cause complete meiotic arrest leading to azoospermia and subsequent infertility. We here unravel two clearly distinct meiotic arrest mechanisms that act during the prophase of human male meiosis. Type I arrested spermatocytes display severe asynapsis of the homologous chromosomes, disturbed XY-body formation and increased expression of the Y-chromosome encoded gene ZFY and seem to activate a DNA damage pathway leading to induction of p63 mediated spermatocyte elimination. Type II arrested spermatocytes display normal chromosome synapsis, normal XY-body morphology and meiotic crossover formation but have a lowered expression of several cell cycle regulating genes and fail to properly silence the X-chromosome encoded gene ZFX. -
Measurement by Quantitative PCR of Changes in HPRT, PGK-1, PGK-2, APRT, Mtase, and Zfy Gene Transcripts During Mouse Spermatogenesis
NucleicNRlAcids Research, Vol. 18, No. 5 1990 Oxford University Press 1255 Measurement by quantitative PCR of changes in HPRT, PGK-1, PGK-2, APRT, MTase, and Zfy gene transcripts during mouse spermatogenesis Judith Singer-Sam, Murray O.Robinson', Anthony R.Bellve2, Melvin l.Simon' and Arthur D.Riggs Division of Biology, Beckman Research Institute of the City of Hope, Duarte, CA 91010, 'Caltech, Division of Biology, Pasadena, CA 91125 and 2Departments of Anatomy and Cell Biology, Urology and the Center for Reproductive Sciences, College of Physicians and Surgeons, Columbia University, New York, NY 10032, USA Received October 12, 1989; Revised and Accepted February 6, 1990 ABSTRACT A reverse transcriptase-polymerase chain reaction genes, PGK-1 and HPRT, the Y-linked Zfy genes were also assay (RT-PCR) was used quantitatively to measure included in the study because they may be involved in accumulated levels of RNA transcripts in total mouse spermatogenesis (12). RNAs derived from male germ cells at various Three other genes were investigated. The autosomal gene for spermatogenic stages. RNA levels for two X-linked adenine phosphoribosyltransferase (APRT), was included in the enzymes, phosphoglycerate kinase (PGK-1) and study because, like HPRT, APRT is part of the purine salvage hypoxanthine phosphoribosyl transferase (HPRT), both pathway and is expressed ubiquitiously at low levels. decrease during spermatogenesis, although the Phosphoglycerate kinase-2 (PGK-2), an autosomal, testis-specific transcript levels decrease much more rapidly for isozyme of PGK-1, was included as a control because it is known PGK-1. RNA for the Y-linked ZFY (zinc finger protein) to be expressed only in meiotic and post-meiotic male germ cells is elevated in all spermatogenic cell fractions tested, (13). -
DEAH)/RNA Helicase a Helicases Sense Microbial DNA in Human Plasmacytoid Dendritic Cells
Aspartate-glutamate-alanine-histidine box motif (DEAH)/RNA helicase A helicases sense microbial DNA in human plasmacytoid dendritic cells Taeil Kima, Shwetha Pazhoora, Musheng Baoa, Zhiqiang Zhanga, Shino Hanabuchia, Valeria Facchinettia, Laura Bovera, Joel Plumasb, Laurence Chaperotb, Jun Qinc, and Yong-Jun Liua,1 aDepartment of Immunology, Center for Cancer Immunology Research, University of Texas M. D. Anderson Cancer Center, Houston, TX 77030; bDepartment of Research and Development, Etablissement Français du Sang Rhône-Alpes Grenoble, 38701 La Tronche, France; and cDepartment of Biochemistry, Baylor College of Medicine, Houston, TX 77030 Edited by Ralph M. Steinman, The Rockefeller University, New York, NY, and approved July 14, 2010 (received for review May 10, 2010) Toll-like receptor 9 (TLR9) senses microbial DNA and triggers type I Microbial nucleic acids, including their genomic DNA/RNA IFN responses in plasmacytoid dendritic cells (pDCs). Previous and replicating intermediates, work as strong PAMPs (13), so studies suggest the presence of myeloid differentiation primary finding PRR-sensing pathogenic nucleic acids and investigating response gene 88 (MyD88)-dependent DNA sensors other than their signaling pathway is of general interest. Cytosolic RNA is TLR9 in pDCs. Using MS, we investigated C-phosphate-G (CpG)- recognized by RLRs, including RIG-I, melanoma differentiation- binding proteins from human pDCs, pDC-cell lines, and interferon associated gene 5 (MDA5), and laboratory of genetics and physi- regulatory factor 7 (IRF7)-expressing B-cell lines. CpG-A selectively ology 2 (LGP2). RIG-I senses 5′-triphosphate dsRNA and ssRNA bound the aspartate-glutamate-any amino acid-aspartate/histi- or short dsRNA with blunt ends.